7.3: Biogeochemical Cycles
( \newcommand{\kernel}{\mathrm{null}\,}\)
Biogeochemical cycles, also known as nutrient cycles, describe the movement of chemical elements through different media, such as the atmosphere, soil, rocks, bodies of water, and organisms. Biogeochemical cycles keep essential elements available to plants and other organisms.
Energy flows directionally through ecosystems, entering as sunlight (or inorganic molecules for chemoautotrophs) and leaving as heat during energy transformation between trophic levels. Rather than flowing through an ecosystem, the matter that makes up organisms is conserved and recycled. The law of conservation of mass states that matter is neither created nor destroyed. For example, after a chemical reaction, the mass of the products (ending molecules) will be the same as the mass of the reactants (starting molecules). The same is true in an ecosystem. Matter moves through different media, and atoms may react to form new molecules, but the amount of matter remains constant.
The biogeochemical cycles of four elements—carbon, nitrogen, phosphorus, and sulfur—are discussed below. The cycling of these elements is interconnected with the water cycle. For example, the movement of water is critical for the leaching of sulfur and phosphorus into rivers, lakes, and oceans. Today, anthropogenic (human) activities are altering all major ecosystems and the biogeochemical cycles they drive.
The Carbon Cycle
Carbon is the basic building block of all organic materials, and therefore, of living organisms. The carbon cycle is actually comprised of several interconnected cycles: one dealing with rapid carbon exchange among living organisms and the other dealing with the long-term cycling of carbon through geologic processes (figure 7.3.a). The overall effect is that carbon is constantly recycled in the dynamic processes taking place in the atmosphere, at the surface and in the crust of the earth. The vast majority of carbon resides as inorganic minerals in crustal rocks. Other reservoirs of carbon, places where carbon accumulates, include the oceans and atmosphere. Some of the carbon atoms in your body today may long ago have resided in a dinosaur's body, or perhaps were once buried deep in the Earth's crust as carbonate rock minerals.
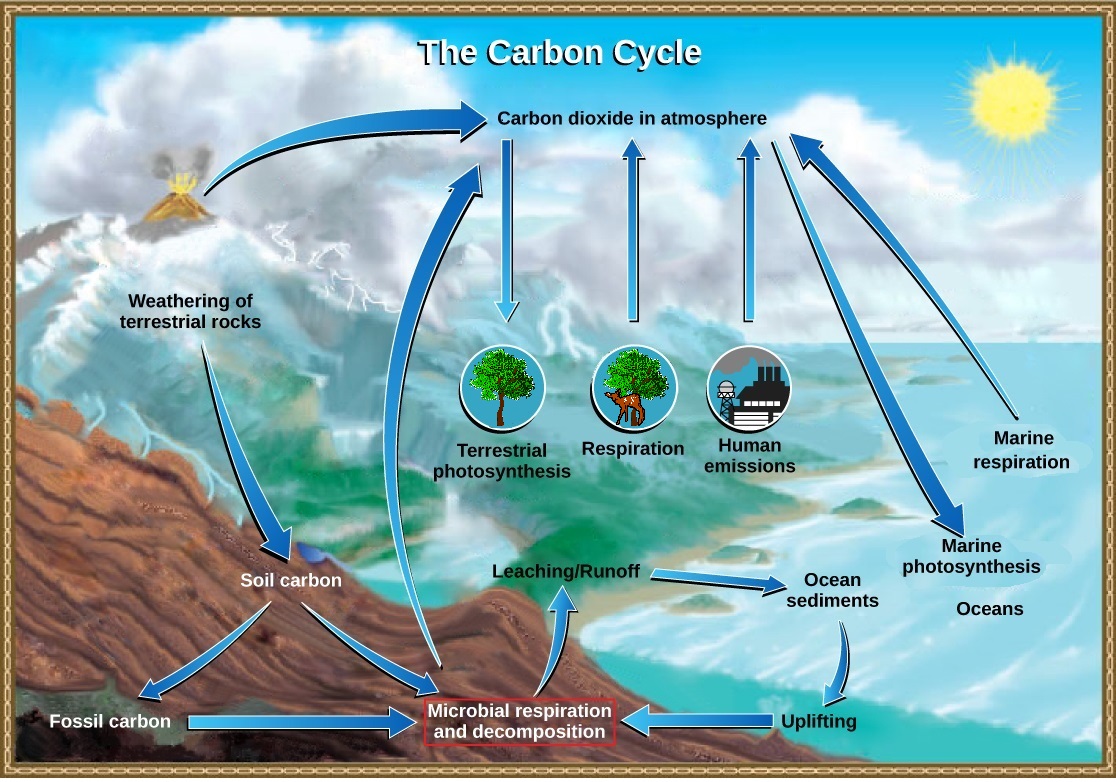
Carbon Cycles Slowly between Land and the Ocean
On land, carbon is stored in soil as organic carbon in the form of decomposing organisms or terrestrial rocks. Decomposed plants and algae are sometimes buried and compressed between layers of sediments. After millions of years fossil fuels such as coal, oil, and natural gas are formed. The weathering of terrestrial rock and minerals release carbon into the soil.
Carbon-containing compounds in the soil can be washed into bodies of water through leaching. This water eventually enters the ocean. Atmospheric carbon dioxide also dissolves in the ocean, reacting with water molecules to form carbonate ions (CO32-). Some of these ions combine with calcium ions in the seawater to form calcium carbonate (CaCO3), a major component of the shells of marine organisms. These organisms eventually die and their shells form sediments on the ocean floor. Over geologic time, the calcium carbonate forms limestone, which comprises the largest carbon reservoir on Earth.
Carbonate also precipitates in sediments, forming carbonate rocks, such as limestone. Carbon sediments from the ocean floor are taken deep within Earth by the process of subduction: the movement of one tectonic plate beneath another. The ocean sediments are subducted by the actions of plate tectonics, melted and then returned to the surface during volcanic activity. Plate tectonics can also cause uplifting, returning ocean sediments to land.
Carbon Cycles Quickly between Organisms and the Atmosphere
Carbon dioxide is converted into glucose, an energy-rich organic molecule through photosynthesis by plants, algae, and some bacteria (figure 7.3.b). They can then produce other organic molecules like complex carbohydrates (such as starch), proteins and lipids, which animals can eat. Most terrestrial autotrophs obtain their carbon dioxide directly from the atmosphere, while marine autotrophs acquire it in the dissolved form (bicarbonate, HCO3–).
Plants, animals, and other organisms break down these organic molecules during the process of aerobic cellular respiration, which consumes oxygen and releases energy, water and carbon dioxide. Carbon dioxide is returned to the atmosphere during gaseous exchange. Another process by which organic material is recycled is the decomposition of dead organisms. During this process, bacteria and fungi break down the complex organic compounds. Decomposers may do respiration, releasing carbon dioxide, or other processes that release methane (CH4).
Photosynthesis and respiration are actually reciprocal to one another with regard to the cycling of carbon: photosynthesis removes carbon dioxide from the atmosphere and respiration returns it (figure 7.3.c). A significant disruption of one process can therefore affect the amount of carbon dioxide in the atmosphere.
Cellular respiration is only one process that releases carbon dioxide. Physical processes, such as the eruption of volcanoes and release from hydrothermal vents (openings in the ocean floor) add carbon dioxide to the atmosphere. Additionally, the combustion of wood and fossil fuels releases carbon dioxide. The level of carbon dioxide in the atmosphere is greatly influenced by the reservoir of carbon in the oceans. The exchange of carbon between the atmosphere and water reservoirs influences how much carbon is found in each.
Importance of the Carbon Cycle
The carbon cycle is crucially important to the biosphere. If not for the recycling processes, carbon might long ago have become completely sequestered in crustal rocks and sediments, and life would no longer exist (figure 7.3.e). Photosynthesis not only makes energy and carbon available to higher trophic levels, but it also releases gaseous oxygen (O2). Gaseous oxygen is necessary for cellular respiration to occur. Photosynthetic bacteria were likely the first organisms to perform photosynthesis, dating back 2-3 billion years ago. Thanks to their activity, and a diversity of present-day photosynthesizing organisms, Earth’s atmosphere is currently about 21% O2. Also, this O2 is vital for the creation of the ozone layer, which protects life from harmful ultraviolet radiation emitted by the sun. Ozone (O3) is created from the breakdown and reassembly of O2.
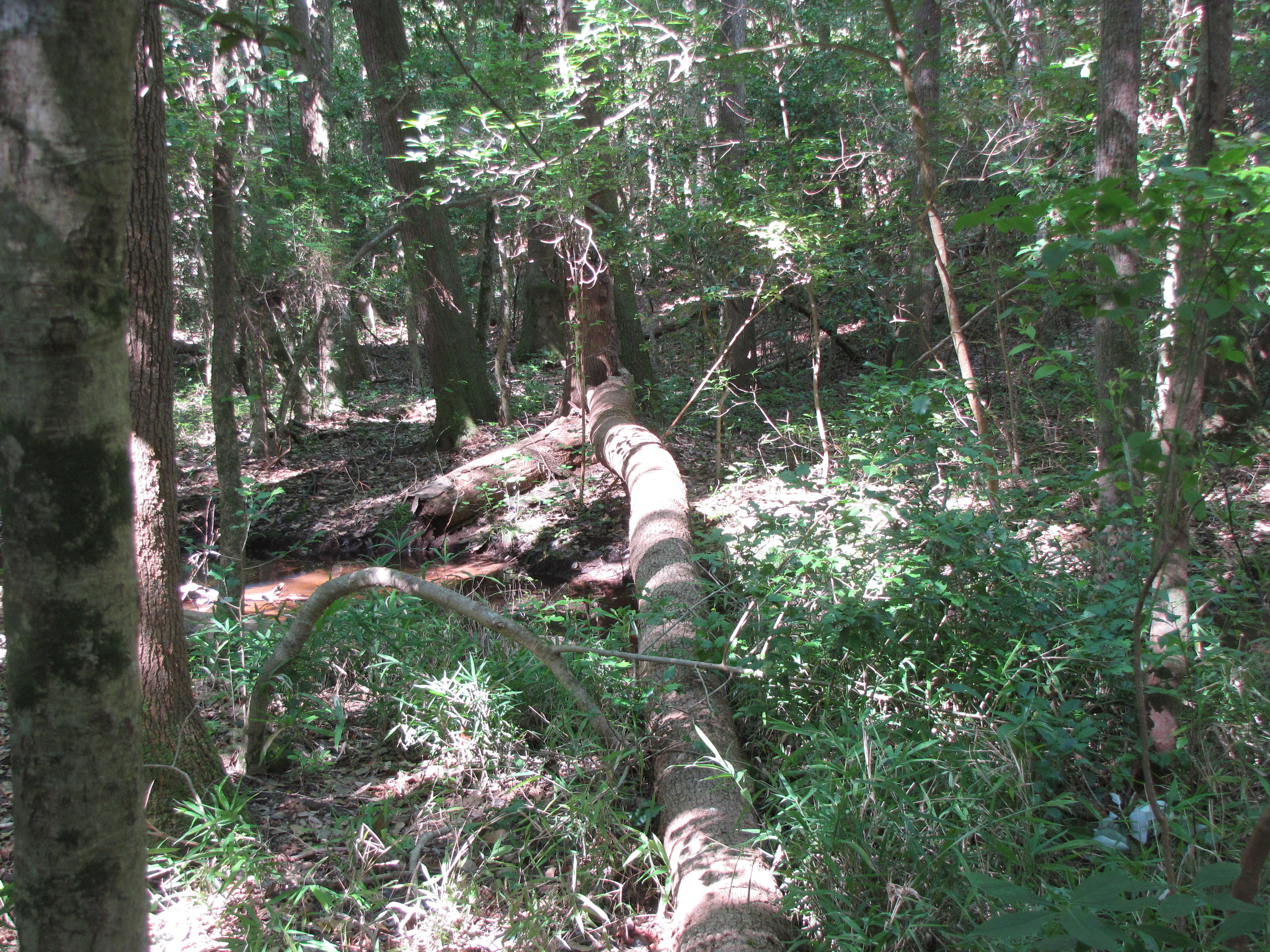
The global carbon cycle contributes substantially to the provisioning ecosystem services upon which humans depend. We harvest approximately 25% of the total plant biomass that is produced each year on the land surface to supply food, fuel wood and fiber from croplands, pastures and forests. In addition, the global carbon cycle plays a key role in regulating ecosystem services because it significantly influences climate via its effects on atmospheric CO2 concentrations.
Human Alteration of the Carbon Cycle
Atmospheric CO2 concentration increased from 280 parts per million (ppm) to 413 ppm between the start of industrial revolution in the late eighteenth century and 2020. This reflected a new flux in the global carbon cycle—anthropogenic CO2 emissions—where humans release CO2 into the atmosphere by burning fossil fuels and changing land use. Fossil fuel burning takes carbon from coal, gas, and oil reserves, where it would be otherwise stored on very long time scales, and introduces it into the active carbon cycle. Land use change releases carbon from soil and plant biomass pools into the atmosphere, particularly through the process of deforestation for wood extraction or conversion of land to agriculture. In 2018, the additional flux of carbon into the atmosphere from anthropogenic sources was estimated to be 36.6 gigatons of carbon (GtC = 1 billion tons of carbon)—a significant disturbance to the natural carbon cycle that had been in balance for several thousand years previously. High levels of carbon dioxide in the atmosphere cause warming that results in climate change. (See Threats to Biodiversity and Climate Change for more details.)
The Nitrogen Cycle
All organisms require nitrogen because it is an important component of nucleic acids, proteins, and other organic molecules. Getting nitrogen into living organisms is difficult. Plants and algae are not equipped to incorporate nitrogen from the atmosphere (where it exists as tightly bonded, triple covalent N2) although this molecule comprises approximately 78 percent of the atmosphere. Because most of the nitrogen is stored in the atmosphere, the atmosphere is considered a reservoir of nitrogen.
The nitrogen molecule (N2) is quite inert. To break it apart so that its atoms can combine with other atoms requires the input of substantial amounts of energy. Nitrogen fixation is the process of converting nitrogen gas into ammonia (NH3), which spontaneously becomes ammonium (NH4+). Ammonium is found in bodies of water and in the soil (figure 7.3.f).
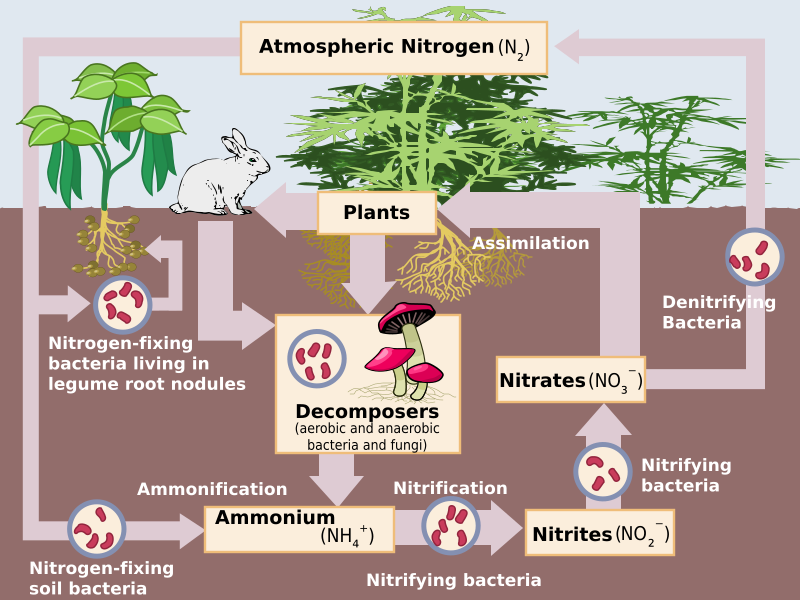
Three processes are responsible for most of the nitrogen fixation in the biosphere. The first is atmospheric fixation by lightning. The enormous energy of lightning breaks nitrogen molecules and enables their atoms to combine with oxygen in the air forming nitrogen oxides. These dissolve in rain, forming nitrates, that are carried to the earth. Atmospheric nitrogen fixation probably contributes some 5-8% of the total nitrogen fixed. The second process is industrial fixation. Under great pressure, at a temperature of 600°C (1112°F), and with the use of a catalyst (which facilitates chemical reactions), atmospheric nitrogen and hydrogen can be combined to form ammonia (NH3). Ammonia can be used directly as fertilizer, but most of it is further processed to urea and ammonium nitrate (NH4NO3).
The third process is biological fixation by certain free-living or symbiotic bacteria. Some form a symbiotic relationship with plants in the legume family, which includes beans, peas, soybeans, alfalfa, and clovers (figure 7.3.g). Some nitrogen-fixing bacteria even establish symbiotic relationships with animals, e.g., termites and "shipworms" (wood-eating bivalves). Nitrogen-fixing cyanobacteria are essential to maintaining the fertility of semi-aquatic environments like rice paddies. Although the first stable product of the process is ammonia, this is quickly incorporated into protein and other organic nitrogen compounds.
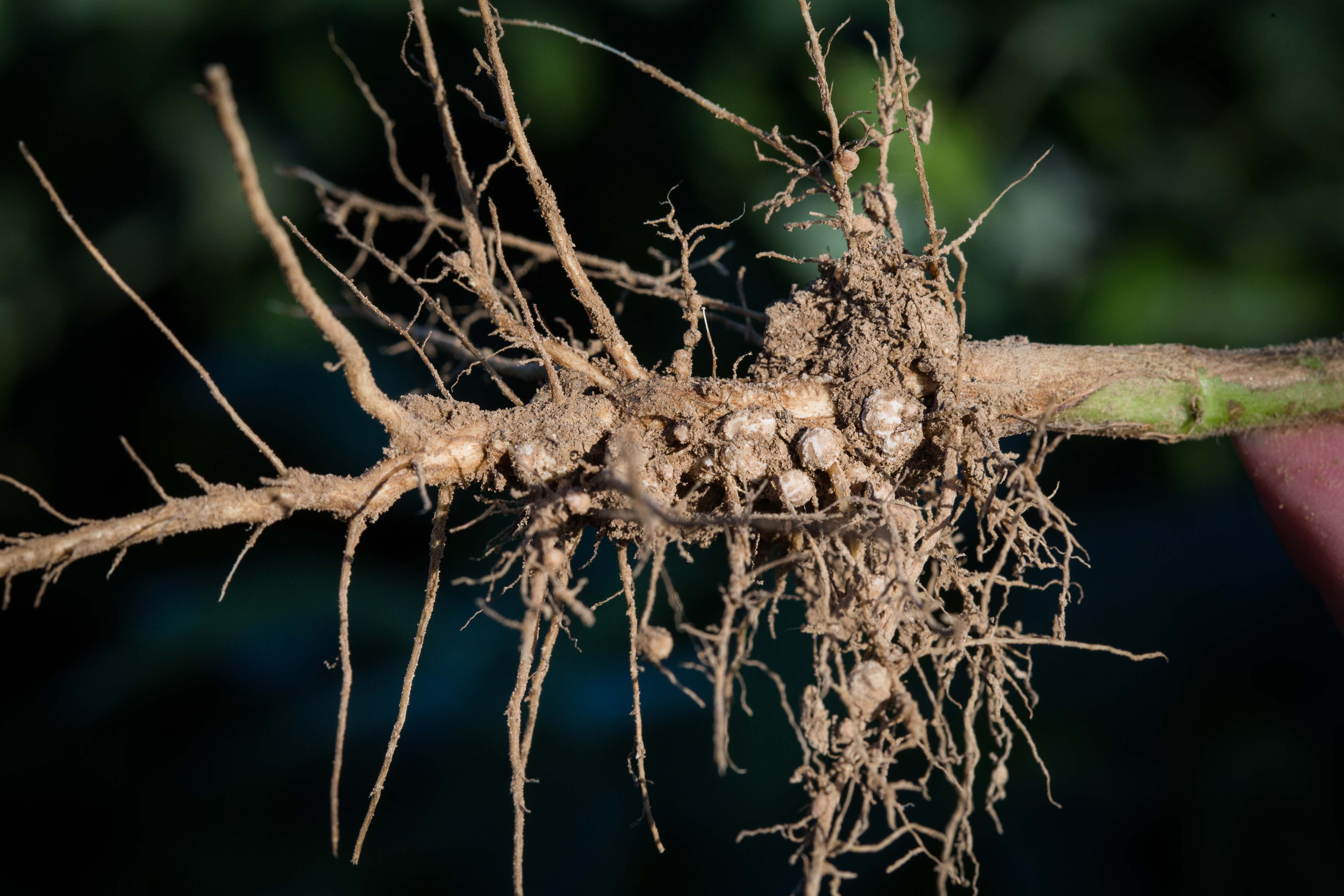
Ammonium is converted by bacteria and archaea into nitrites (NO2−) and then nitrates (NO3−) through the process of nitrification. Like ammonium, nitrites and nitrates are found in water and the soil. Some nitrates are converted back into nitrogen gas, which is released into the atmosphere. The process, called denitrification, is conducted by bacteria.
Plants and other producers directly use ammonium and nitrates to make organic molecules through the process of assimilation. This nitrogen is now available to consumers. Organic nitrogen is especially important to the study of ecosystem dynamics because many processes, such as primary production, are limited by the available supply of nitrogen.
Consumers excrete organic nitrogen compounds that return to the environment. Additionally dead organisms at each trophic level contain organic nitrogen. Microorganisms, such as bacteria and fungi, decompose these wastes and dead tissues, ultimately producing ammonium through the process of ammonification.
In marine ecosystems, nitrogen compounds created by bacteria, or through decomposition, collects in ocean floor sediments. It can then be moved to land in geologic time by uplift of Earth’s crust and thereby incorporated into terrestrial rock. Although the movement of nitrogen from rock directly into living systems has been traditionally seen as insignificant compared with nitrogen fixed from the atmosphere, a recent study showed that this process may indeed be significant and should be included in any study of the global nitrogen cycle.
Human activity can alter the nitrogen cycle by two primary means: the combustion of fossil fuels, which releases different nitrogen oxides into the atmosphere, and by the use of artificial fertilizers in agriculture. Atmospheric nitrogen (other than N2) is associated with several effects on Earth’s ecosystems. Nitrogen oxides (HNO3) can react in the atmosphere to form nitric acid, a form of acid deposition, also known as acid rain. Acid deposition damages healthy trees, destroys aquatic systems and erodes building materials such as marble and limestone. Like carbon dioxide, nitrous oxide (N2O) causes warming resulting in climate change.
Humans are primarily dependent on the nitrogen cycle as a supporting ecosystem service for crop and forest productivity. Nitrogen fertilizers are added to enhance the growth of many crops and plantations (figure 7.3.h). The enhanced use of fertilizers in agriculture was a key feature of the green revolution that boosted global crop yields in the 1970s. The industrial production of nitrogen-rich fertilizers has increased substantially over time and now matches more than half of the input to the land from biological nitrogen fixation (90 megatons = 1 million tons of nitrogen each year). If the nitrogen fixation from legume crops is included, then the anthropogenic flux of nitrogen from the atmosphere to the land exceeds natural fluxes to the land. Fertilizers are washed into lakes, streams, and rivers by surface runoff, resulting in saltwater and freshwater eutrophication, a process whereby nutrient runoff causes the overgrowth of algae, the depletion of oxygen, and death of aquatic fauna.
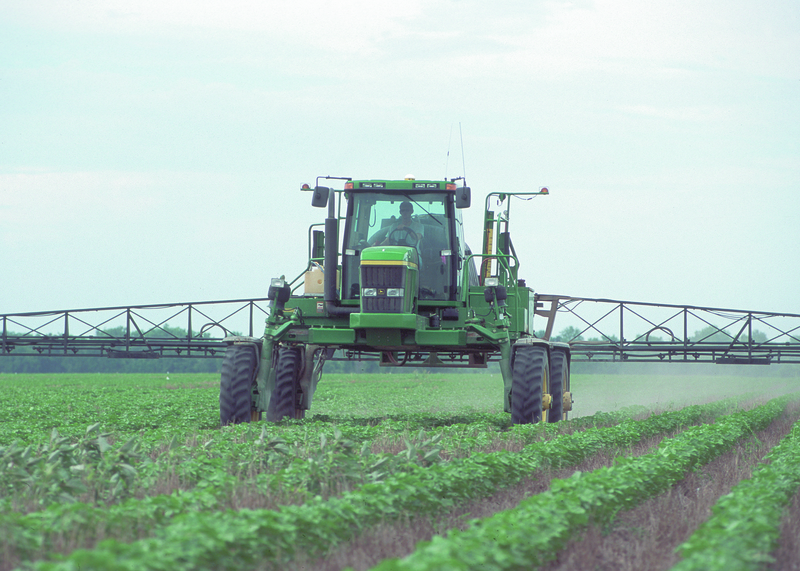
The Phosphorus Cycle
Several forms of nitrogen (nitrogen gas, ammnoium, nitrates, etc.) were involved in the nitrogen cycle, but phosphorus remains primarily in the form of the phosphate ion (PO43-). Also in contrast to the nitrogen cycle, there is no form of phosphorus in the atmosphere. Phosphorus is used to make nucleic acids and the phospholipids that comprise biological membranes.
Rocks are a reservoir for phosphorus, and these rocks have their origins in the ocean. Phosphate-containing ocean sediments form primarily from the bodies of ocean organisms and from their excretions. However, volcanic ash, aerosols, and mineral dust may also be significant phosphate sources. This sediment then is moved to land over geologic time by the uplifting of Earth’s surface (figure 7.3.i). The movement of phosphate from the ocean to the land and through the soil is extremely slow, with the average phosphate ion having an oceanic residence time between 20,000 and 100,000 years.
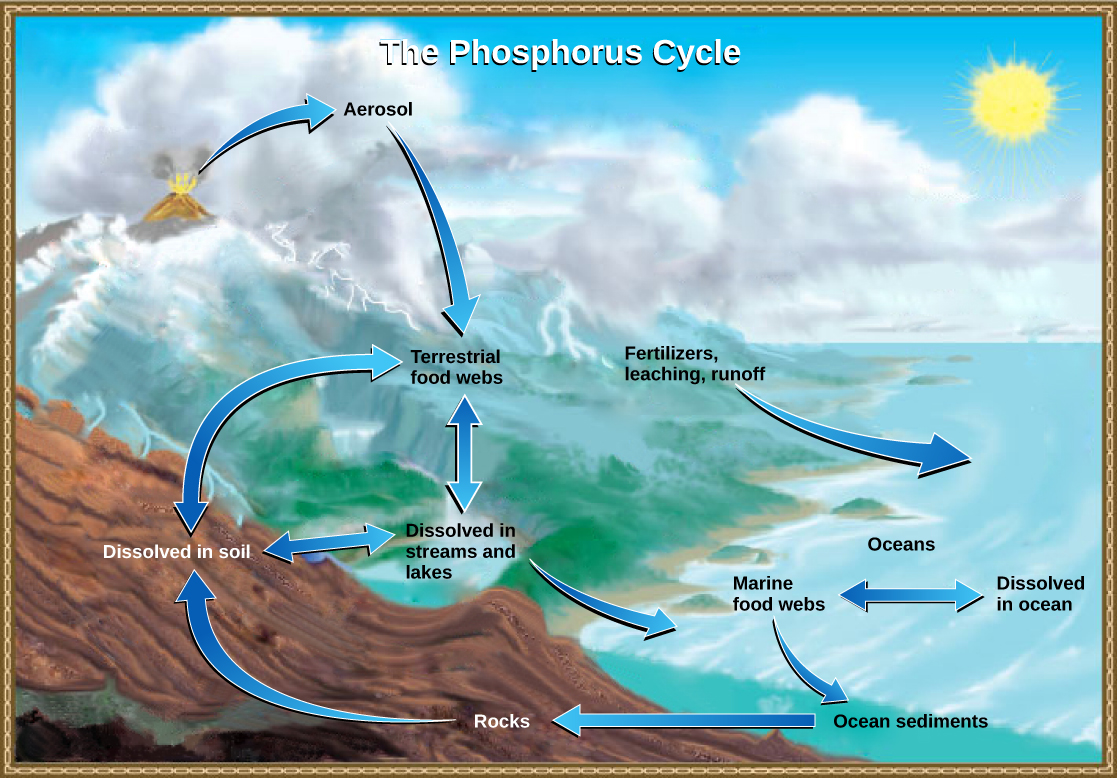
Marine birds play a unique role in the phosphorous cycle. These birds take up phosphorous from ocean fish. Their droppings on land (guano) contain high levels of phosphorous and are sometimes mined for commercial use. A 2020 study estimated that the ecosystem services (natural processes and products that benefit humans) provided by guano are worth $470 million per year.
Weathering of rocks releases phosphates into the soil and bodies of water. Plants can assimilate phosphates in the soil and incorporate it into organic molecules, making phosphorus available to consumers in terrestrial food webs. Waste and dead organisms are decomposed by fungi and bacteria, releasing phosphates back into the soil. Some phosphate is leached from the soil, entering into rivers, lakes, and the ocean. Primary producers in aquatic food webs, such as algae and photosynthetic bacteria, assimilate phosphate, and organic phosphate is thus available to consumers in aquatic food webs. Similar to terrestrial food webs, phosphorus is reciprocally exchanged between phosphate dissolved in the ocean and organic phosphorus in marine organisms.
The movement of phosphorus from rock to living organisms is normally a very slow process, but some human activities speed up the process. Phosphate-bearing rock is often mined for use in the manufacture of fertilizers and detergents. This commercial production greatly accelerates the phosphorous cycle. In addition, runoff from agricultural land and the release of sewage into water systems can cause a local overload of phosphate. The increased availability of phosphate can cause overgrowth of algae. This reduces the oxygen level, causing eutrophication and the destruction of other aquatic species.
Eutrophication and Dead Zones
Eutrophication occurs when excess phosphorus and nitrogen from fertilizer runoff or sewage causes excessive growth of algae. Algal blooms that block light and therefore kill aquatic plants in rivers, lakes, and seas. The subsequent death and decay of these organisms depletes dissolved oxygen, which leads to the death of aquatic organisms such as shellfish and fish. This process is responsible for dead zones, large areas in lakes and oceans near the mouths of rivers that are periodically depleted of their normal flora and fauna, and for massive fish kills, which often occur during the summer months (figure 7.3.j). There are more than 500 dead zones worldwide. One of the worst dead zones is off the coast of the United States in the Gulf of Mexico. Fertilizer runoff from the Mississippi River basin created a dead zone, which reached its peak size of 8,776 square miles in 2017. Phosphate and nitrate runoff from fertilizers also negatively affect several lake and bay ecosystems including the Chesapeake Bay in the eastern United States.
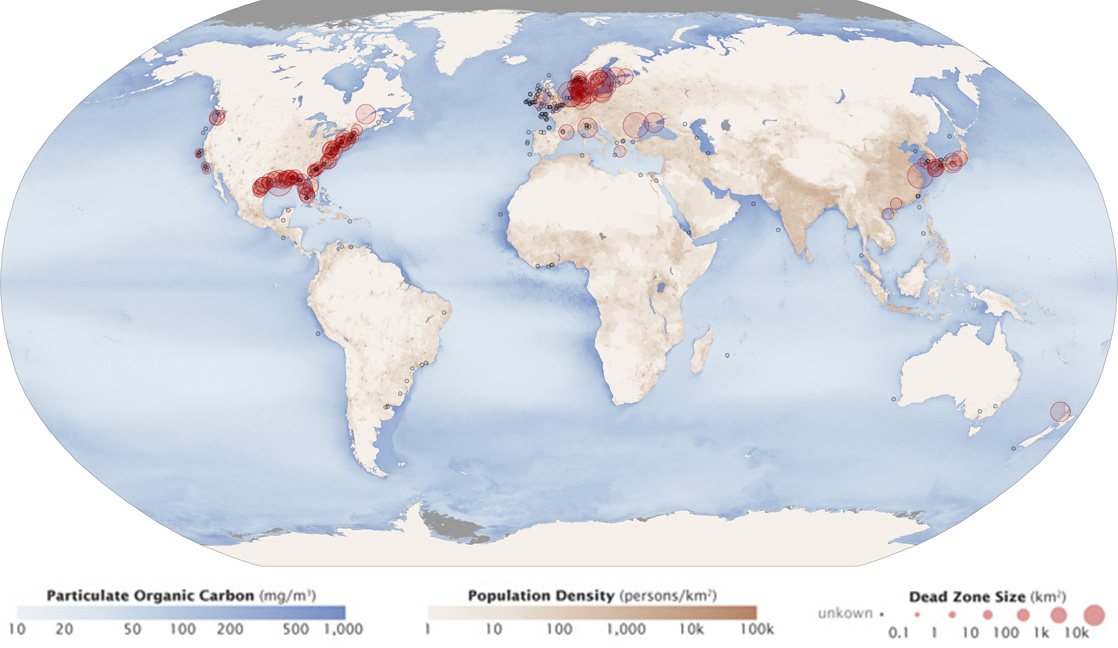
Everyday Connection: Chesapeake Bay
The Chesapeake Bay has long been valued as one of the most scenic areas on Earth; it is now in distress and is recognized as a declining ecosystem. In the 1970s, the Chesapeake Bay was one of the first ecosystems to have identified dead zones, which continue to kill many fish and bottom-dwelling species, such as clams, oysters, and worms (figure 7.3.k). Several species have declined in the Chesapeake Bay due to surface water runoff containing excess nutrients from artificial fertilizer used on land. The source of the fertilizers (with high nitrogen and phosphate content) is not limited to agricultural practices. There are many nearby urban areas and more than 150 rivers and streams empty into the bay that are carrying fertilizer runoff from lawns and gardens. Thus, the decline of the Chesapeake Bay is a complex issue and requires the cooperation of industry, agriculture, and everyday homeowners.
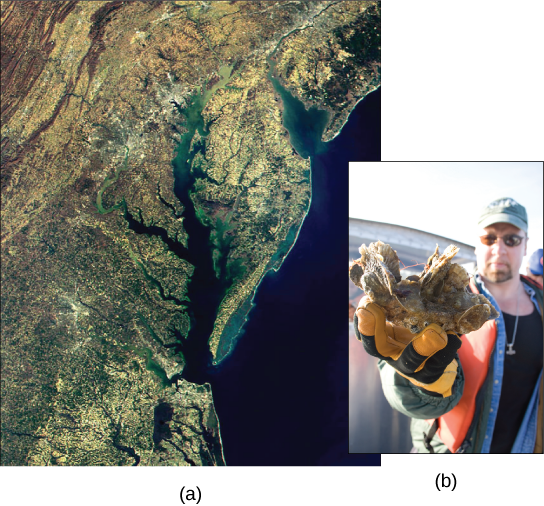
Of particular interest to conservationists is the oyster population; it is estimated that more than 200,000 acres of oyster reefs existed in the bay in the 1700s, but that number has now declined to only 36,000 acres. Oyster harvesting was once a major industry for Chesapeake Bay, but it declined 88 percent between 1982 and 2007. This decline was due not only to fertilizer runoff and dead zones but also to overexploitation. Oysters require a certain minimum population density because they must be in close proximity to reproduce. Human activity has altered the oyster population and locations, greatly disrupting the ecosystem.
The restoration of the oyster population in the Chesapeake Bay has been ongoing for several years with mixed success. Not only do many people find oysters good to eat, but they also clean up the bay. Oysters are filter feeders, and as they eat, they clean the water around them. In the 1700s, it was estimated that it took only a few days for the oyster population to filter the entire volume of the bay. Today, with changed water conditions, it is estimated that the present population would take nearly a year to do the same job.
Restoration efforts have been ongoing for several years by non-profit organizations, such as the Chesapeake Bay Foundation. The restoration goal is to find a way to increase population density so the oysters can reproduce more efficiently. Many disease-resistant varieties (developed at the Virginia Institute of Marine Science for the College of William and Mary) are now available and have been used in the construction of experimental oyster reefs. Efforts to clean and restore the bay by Virginia and Delaware have been hampered because much of the pollution entering the bay comes from other states, which stresses the need for inter-state cooperation to gain successful restoration.
The new, hearty oyster strains have also spawned a new and economically viable industry—oyster aquaculture—which not only supplies oysters for food and profit, but also has the added benefit of cleaning the bay.
The Sulfur Cycle
Sulfur is an essential element for the molecules of living things. As part of the amino acid cysteine, it is critical to the three-dimensional shape of proteins. As shown in Figure 7.3.l, sulfur cycles among the oceans, land, and atmosphere. Atmospheric sulfur is found in the form of sulfur dioxide (SO2), which enters the atmosphere in three ways: first, from the decomposition of organic molecules; second, from volcanic activity and geothermal vents; and, third, from the burning of fossil fuels by humans.
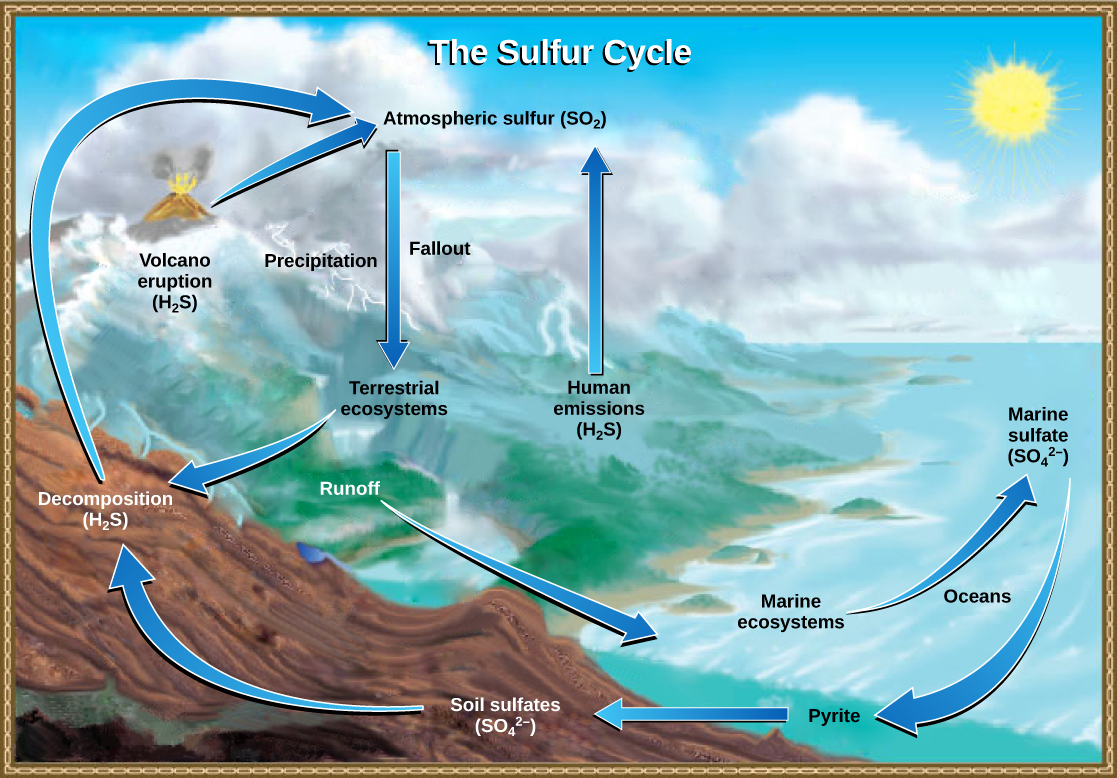
On land, sulfur is deposited in four major ways: precipitation, direct fallout from the atmosphere, rock weathering, and geothermal vents. Atmospheric sulfur is found in the form of sulfur dioxide (SO2), and as rain falls through the atmosphere, sulfur is dissolved in the form of weak sulfuric acid (H2SO4). Sulfur can also fall directly from the atmosphere in a process called fallout. Also, as sulfur-containing rocks weather, sulfur is released into the soil. These rocks originate from ocean sediments that are moved to land by the geologic uplifting of ocean sediments. Terrestrial ecosystems can then make use of these soil sulfates (SO42-), which enter the food web by being taken up by plant roots. When these plants decompose and die, sulfur is released back into the atmosphere as hydrogen sulfide (H2S) gas.
Sulfur enters the ocean in runoff from land, from atmospheric fallout, and from hydrothermal vents. Some ecosystems rely on microorganisms using sulfur as a biological energy source (in contrast to ecosystems with photosynthetic producers). This sulfur then supports marine ecosystems in the form of sulfates.
Human activities have played a major role in altering the balance of the global sulfur cycle. The burning of large quantities of fossil fuels, especially from coal, releases sulfur dioxide, which reacts with the atmosphere to form sulfuric acid. Like nitric acid, sulfuric acid contributes to acid deposition.
Suggested Supplementary Reading
Bruckner, M. 2018. The Gulf of Mexico Dead Zone. [Website]
References
Cell Press. (2020, August 6). Researchers hope to save seabirds by calculating the value of their excrement. Retrieved August 7, 2020 from ScienceDaily.
Attributions
Modified by Melissa Ha from the following sources:
- Biogeochemical Cycles, Energy, and Energy Enters Ecosystems Through Photosynthesis from Environmental Biology by Matthew R. Fisher (licensed under CC-BY)
- Carbon Cycle and Nitrogen Cycle from Biology by John W. Kimball (licensed under CC-BY)
- Energy Flow through Ecosystems from General Biology by OpenStax (licensed under CC-BY)
- Soil and Sustainability and Biogeochemical Cycles and the Flow of Energy in the Earth System from Sustainability: A Comprehensive Foundation by Tom Theis and Jonathan Tomkin, Editors (licensed under CC-BY). Download for free at CNX.
- Cycling of Matter from AP Environmental Science by University of California College Prep, University of California (licensed under CC-BY). Download for free at CNX.
- Nutrient Cycles from Life Sciences Grade 10 by Siyavula (CC-BY)