10.5: Gene Duplication and Folivory (leaf eating)
- Page ID
- 128946
Gene Duplication and Folivory: A Novel Trait
Earlier in this book, we considered gene duplication as a genetic mechanism for increasing organismal diversity in evolutionary time. In this case-study, we will examine duplication of RNase genes associated with the evolution of leaf eating (folivory) and gut fermentation. Many mammalian clades have evolved gut fermentation and folivory independently, Monkeys have likely evolved it in parallel many times, perhaps not surprisingly given their arboreal lifestyle. Efficient gut fermentation allows monkeys exploit new food resources, leaves, which opens up a new ecological niche. In this way, not only does the complexity of the organism increase (more genes, novel physiological function, new food resource), but so does the complexity of the ecosystem with new ecosystem interactions now possible.
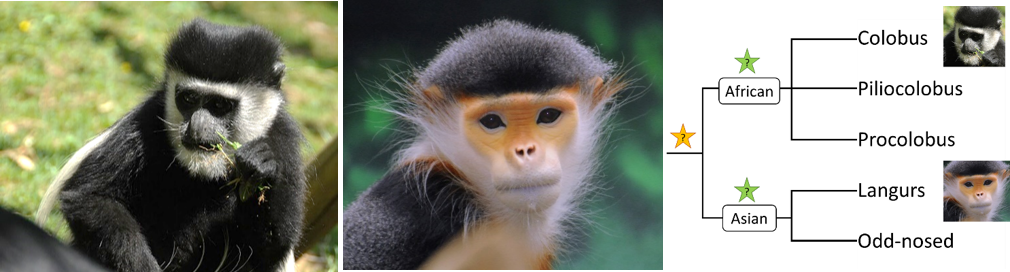
Figure 1: Left: A guereza colobine(Colobus guereza) monkey from Africa eating leaves. Photo credit: Thomas Bresson, originally published on Wikimedia Commons under a CC BY 3.0 license Middle: A douc langur colobine monkey from Asia may have independently evolved folivory. Photo credit: Ucumari photography originally published on Flickr under a CC BY-NC-ND 2.0 license Right: a phylogeny of the colobine monkeys. RNase duplication may have occurred once (yellow star) or twice (green stars), an expanded dataset favors the green star scenario.
Leaves are abundant, why don’t all animals eat them?
Leaves (including blades of grass) have abundant energy-rich carbohydrates, but these carbohydrates are largely inaccessible to animal guts. Unlike carbs in roots and fruits, leaf carbohydrates are largely stored as the structural polysaccharides cellulose and hemicellulose. These have β-1,4-glycosidic linkages between their glucose monomers that animal digestive systems cannot break down. Despite this, many animals like colobine monkeys and cows get much of their energy from digesting leaves. Behavioral, physiological, and anatomical adaptations make this possible. For example, some folivorous animals are known to rest more than their relatives (like koalas) and some use their senses to choose leaves with a higher protein to fiber content (like howler monkeys).
Folivorous animals are also able to extract more energy from dietary fiber (cellulose) than typical mammals. They do this by hosting symbiotic cellulase-producing bacteria in their guts. These anaerobic bacterial symbionts ferment semi-digested fiber in specialized gut compartments. These gut compartments act as fermentation vats. The side products from fermentation as well as the bacteria themselves can then be used by the animal for nutrients.
These specialized gut structures can take various forms and have evolved several times. For example, koalas and rabbits have independently evolved an enlarged cecum (where a human appendix is located) and colon to house their symbiotic bacteria. Because these adaptations occur near the end of the GI tract, koalas and rabbits are considered to be “hindgut fermenters.” On the other hand, ruminants like cows and giraffes have fermentation vats called rumens directly linked to their esophagus (Figure 2). They are called “foregut fermenters.” Colobine monkeys are similar to ruminants in that they have partitioned stomachs, although their stomachs are not chambered like a ruminant stomach. Colobines additionally have at least some ability to house cellulase-digesting bacteria in their hindguts (Figure 2).
Figure 2: Left: Comparison of the familiar human digestive system with a hindgut digester (rabbit). Fermentation occurs in the elaborated cecum of the small intestine. Some hindgut fermenters have fermentation in the colon as well. Image credit: Dr N B Shridhar originally published on Wikimedia under a CC BY-SA 4.0 International license. Middle: Ruminants, like goats and cows, are foregut fermenters. Ruminants have a separate fermentation vat called the rumen. Food is chewed, passed through the rumen for fermentation, then returns to the mouth for additional chewing. Food then goes through additional stomach processing before entering the small intestine. Image credit: originally published in Open Stax Biology under a CC-BY 4.0 license (authors Connie Rye, Robert Wise, Vladimir Jurukovski, Jean DeSaix, Jung Choi, and Yael Avissar). Right: Colobines are thought to primarily ferment in their sacculated stomach (foregut fermentation) but also house cellulase-producing bacteria in their colon (hindgut fermentation). Unlike ruminants, colobines do not have a completely separate structure for foregut fermatation. Image credit: Liu et al, 2022 published under a CC BY-SA-ND 4.0 license.
Genetic duplication can occur on different scales, as small as an exon duplication to duplication of contiguous genes on a chromosome. There are various mutagenic mechanisms that result in gene duplication including whole-chromosome duplications via nondisjunction, tandem duplications via unequal crossover, retrotransposons, and duplications after rearrangements and repair of breaks. Duplication creates a new set of raw materials that can be tinkered with to take on new functions while the original set maintains its original intended purpose. Neofunctionalization occurs when positive selection acts upon the new duplicated set of genes and one or both gain a new function. If this new function is either fit in the environment or neutral, the duplication may be maintained by evolution.
Why maintain duplicated RNases?
Pancreatic RNases are ribonucleases found extracellularly in vertebrates. They were likely early components of the vertebrate immune system but underwent multiple duplications to form a superfamily with a wide array of properties. Among other things, they are involved in angiogenesis, host defense, and stimulation of the activity of endothelial cells. Fermenting mammals have a particular need for high expression levels of gut RNases because their fermenting bacteria keep much of their nitrogen in RNA. This nitrogen of course initially comes from the food the mammal eats, if the fermenting mammal does not recycle the RNA nitrogen, it will lose much of the nitrogen from its food. Therefore, gut RNases play an important role in nitrogen recycling in fermenting mammals.
In ruminants (like cows), RNaseA (a type of RNase1) specializes in bacterial RNA digestion and is produced in the pancreas then delivered to the small intestine. The ruminant small intestine has a slightly alkaline pH, similar to extracellular fluid. Therefore the duplicated ruminant RNase acts at the same pH as the ancestral mammalian RNase. It has however gained a different function: an enhanced ability to degrade double stranded RNA.
Colobine monkeys have a different problem to solve in their intestines. A high amount of fatty acids released during fermentation makes their intestines slightly acidic. The duplicate colobine gut RNases have high activity at slightly acidic pH, unlike ancestral mammalian RNases. Interestingly, colobine RNases lack a high dsRNA digesting function. Mutational studies suggest that this is because the mutations in ruminants that allowed for this function are precluded by other functional mutations in colobine intestinal RNases. This is a potential example of molecular constraints on protein function: the protein can either have high dsRNA digesting activity or it can work at lower pHs but not both. In both ruminants and colobines we can see a pattern of gene duplication followed by neofunctionalization that likely allowed these fermenting mammals to maintain their ancestral RNase function as well as the gain a novel function in digestion.
Like colobines, howler monkeys are also folivorous and appear to have duplicated pancreatic RNases. Although howler monkeys are hindgut fermenters, they are like colobines in that their fermentation process produces abundant fatty acids and thus a low-pH environment. Perhaps not surprisingly, the multiple species of howler monkeys studied have one copy of RNase1 that is similar to the version found in non-folivorous primates and at least one copy that has undergone rapid evolution and neofunctionalization.
Evidence for duplication of RNases associated with folivory
Because RNases are widely duplicated in the vertebrate lineage, it can be difficult to assess whether they specifically duplicated and underwent neofunctionalization in one specific lineage versus another. For example, if we find that both howler and colobine monkeys each have two copies of RNase1, how would we know whether this duplication occurred in their common ancestor or if it occurred more recently and was maintained by selection for fermentation activity (Box 1)? Two different lines of evidence can be used: First, phylogenetic trees can be made from the sequence data and reconciliation analysis can be performed on the sequence tree (Box 1). Second, researchers can perform direct sequence comparison and site-directed mutagenesis to infer whether key functional mutations are the same in the different lineages.
The figure below compares two scenarios for gene duplication, both of which could explain the pattern of two RNase genes in both the colobine and howler lineages. Green boxes denote lineages with at least some folivorous members.
In Scenario 1 (below), a duplication (purple star) of RNase occurred in the last common ancestor of colobines and howlers. The two RNase sequences were inherited by all descendants of this lineage (purple lines denote second copy) and subsequently lost in the macaques and hominoids. Once the sequences duplicate, each copy follows the species divergence pattern. Note that the purple (duplicate) Gene Tree pattern looks exactly like the anthropoid Species Subtree. Species Tree after Pozzi et al, 2014.
In Scenario 2 (left), separate RNase duplication events occur in the colobine and howler lineages (purple stars) giving rise to lineage-specific duplicates (purple lines on right). In this scenario, the resultant colobine RNases are more similar to each other than to either howler RNase. Whereas in Scenario 1 there was one duplication and 2 loss events, in Scenario 2 there are two duplications and no loss events. From only this information, there is no way to know whether duplications or losses are more common and, therefore, which scenario should be favored.
However, using total evidence (coding and non-coding sequence) of primate RNases, Zhang (2009) ran a phylogenetic analysis and found that howler and colobine RNases did not form a monophyletic clade (a group that shares a common ancestor), as would be expected from Scenario 1. Instead, the howler RNases formed a monophyletic clade with each other, as did the colobine RNases, as predicted by Scenario 2.
For additional information on constructing phylogenetic trees, see:
Molecular Analyses and Modern Phylogenetic Trees
Does evolution repeat itself?
Folivory has evolved many times in mammals, leaves are abundant and found year round in many ecosystems, but molecular and physical adaptations to folivory have only been studied in a handful of mammalian species. Sequence changes to RNases have been associated with shifts to folivory in colobines, artiodactyls (specifically the ruminants), hippos, lemurs, sloths, and howler monkeys. Of these, gene duplication has specifically been studied in colobines, lemurs, ruminants, and howler monkeys. The colobine and howler monkey story is perhaps the most intriguing, because it gives insight into the mechanisms of convergent evolution. Specifically, howler and colobine monkey RNases appear to have convergently evolved the same amino acid changes to adapt to the same nutritional resource (Figure 3, see below for more details). It is unlikely that these changes are homologous because howler and colobine monkeys both have close relatives without these functional changes and without the duplicated copies of RNase (Figure 4).
Figure 3: Convergent amino acid changes in transition to folivory
Common amino acid changes in folivorous primates are mapped onto the Scenario 2 (evidence based) RNase gene tree. Purple branches (colobines and howlers) indicate duplicate sequences. After duplication, one copy (the "standard" copy) retains ancestral function and its sequence is similar to the ancestral sequence. The other copy (the "neofunctionalized" copy) is free to evolve and accumulates mutations. Coding mutations can lead to changes in amino acid sequence. If these sequence changes lead to a more useful (or at least not less useful) protein, then they may be retained (positive selection).
Amino acid changes in folivorous lineages are shown above the lineage line. Changes are included if they are found in at least half of the folivorous species examined from that lineage and are also found in at least one other folivorous lineage. The original position and amino acid code is on the left, the new amino acid at that position is on the right. Note that rapid evolution occurs in only one copy of the colobine and howler lineages. Lemurs, another group of mostly folivorous primates, has only a single copy of RNaseA, but exhibits many of the same amino acid changes as the colobine and howler monkeys.
How often have primate RNases duplicated?
Even though gene duplication may seem like a rare event, its rate in evolutionary history is not well known and likely differs between lineages. While the phylogenetic evidence strongly suggests independent duplications in the howler and colobine lineages (Box 1 and Figure 1), RNase may have also duplicated within the colobines themselves. An analysis comparing douc langur (Pygathrix nemaeus, an African colobine) RNase sequences to guereza (Colobus guereza, an Asian colobine) showed that douc langur had two RNase1 (pancreatic RNase) genes and guereza had three. Each species maintained a “typical” RNase1 with similar sequence and optimal pH to Rhesus monkey and human RNase1. The “atypical” RNase1s all showed signs of positive selection, had a lower optimal pH, and had lost the ability to efficiently degrade dsRNA.
All of the neofunctionalized proteins have amino acid substitutions that give them the lower optimal pH (and lower their isoelectric point). At first glance this might suggest that these proteins originated in a duplication event in an ancestral colobine. However, another 10 amino acid substitutions differ between the two species. Phylogenetic analysis using whole sequences gave ambiguous results depending on the methodology. This could be due to the accelerated evolution in the neofunctionalized proteins. However, the non-coding sequences do not show accelerated evolution. When these were used in a phylogenetic analysis examining 12 colobine species, all African colobine pancreatic RNase sequences fell into a single moderately-well supported clade to the exclusion of the Asian colobine sequences. Whole-sequence analysis produced well supported Asian and African clades (Figure 5). If a duplication occurred in an ancestral colobine, the ancestral colobine RNase1 sequences should fall in a single clade to the exclusion of the neofunctionalized sequences.
Figure 4: Comparison of 9 key adaptive sites in primate RNase1s. Other studies have shows that most primate RNase1s are very similar to the human ortholog (top row, dots) at the illustrated residues, suggesting purifying selection. Folivorous lemurs (green highlights) do not have duplicated RNase1 genes. However, their single copy shows accelerated evolution. The new copies of RNase1 in colobines and howler monkeys (grey highlights) show accelerated evolution at the same sites as lemur RNase1s. The non-neofunctionalized colobine and howler RNase1s are very similar to the human, macaque, and chimp sequences.
Folivorous species are indicated with a green leaf. Residues are color coded by function. pI indicates the estimated isoelectric point of each protein. Higher pIs are found in proteins that are more positively charged at a neutral pH. Lemur RNases and the nonfunctionalized colobine and howler RNases tend to exhibit lower pIs than RNases with ancestral sequences. This may be associated with their novel function in a more acidic (low pH) environment.
Originally published in Guevara, Greene, et al, 2021 under a CC BY license.
Multiple parallel evolution in primates
Because of the similarities in both fermentation and gut environments between colobines and howlers, researchers compared the neofunctionalized RNase1 sequences between these clades. When compared to cow and human RNase1 sequence, all monkey sequences were more similar to humans This suggests there was not sequence convergence between cows and monkeys to support bacterial fermentation. When comparing all primate sequences, the non-neofunctionalized RNases are very similar to each other and to the human sequence. The neofunctionalized proteins, however, have some specific residues in common, even across clades. For example all neofunctionalized RNases and only 2 standard RNases show a conversion of R ->W at residue 39 (human sequence). Many neofunctionalized RNases show conversion of R->Q at residue 4 and K->E at residue 6, these residues are not found in any of the standard sequences (Figures 3 and 4). Because howler monkeys and colobines are so evolutionarily distant and their closer relatives lack these changes, these novel proteins represent convergent evolution of both function and sequence.
Neofunctionalization without duplication?
Lemurs, non-monkey primates, also have several folivorous lineages, which are hindgut fermenters like howler monkeys. However, unlike howlers and colobines there is no evidence of RNase duplication in the lemurs. However, the single pancreatic RNase in lemur genomes has undergone rapid evolution at the same sites seen in the neofunctionalized colobine and howler RNases (Figures 3 and 4). Several of these sites were predicted to affect catalytic activity in other primates. In particular, the 83D->E substitution found in colobines and known to greatly affect RNase activity is also found in the single-copy sifaka lemur sequences, though intriguingly not in other folivorous lemur sequences. It is possible that recent evolution of folivory, dietary flexibility, or even non-involvement of RNase with folivory in lemurs is responsible for this pattern and the lack of duplicate genes.
Other RNase expansions
Carnivora (Felines, bears, canids, etc.) , a class with a short and simple digestive system compared to other mammals, has also had multiple gene expansions within the Mustelids (ferrets, badgers, wolverines, etc.). Carnivora do not typically have a great deal of microbial digestion because they largely eat meat, which is fully digestible by native enzymes. Molecular and computational analysis suggest that these expansions are associated with novel physiological functions. Gene expansions of RNase1 in insectivorous bats are thought to be associated with immune, rather than digestive functions. Intriguingly, bat lineages with other feeding patterns did not show expansion.
Summary
Leaf eating (folivory) has evolved numerous times in mammalian lineages. The evolution of a fermentation vat in the gut that houses cellulose-digesting bacteria comes along with a cost: loss of nitrogen to bacterial RNA. Several lineages have duplicated and neofunctionalized RNases to recycle this nitrogen back into the organism instead of losing it as waste. This duplication has occurred convergently in howler monkeys and colobine monkeys. Additionally, there is some evidence to suggest that it has happened at least twice in colobine lineages. The evidence for convergent evolution comes from comparing both coding and non-coding gene sequences. The coding gene sequences show some convergent amino-acid substitutions in sites that affect the isoelectric point and enzymatic activity of the RNases. More recently evolved is folivory in lemurs (non-monkey primates). The lemur single RNases show accelerated evolution but not duplication. Future work will likely examine additional folivorous groups as well as the function of RNases in groups with gene expansions for non-digestive functions.
References
Barker, Christopher J., Amber Gillett, Adam Polkinghorne, and Peter Timms. “Investigation of the Koala (Phascolarctos Cinereus) Hindgut Microbiome via 16S Pyrosequencing.” Veterinary Microbiology 167, no. 3–4 (December 2013): 554–64. https://doi.org/10.1016/j.vetmic.2013.08.025.
Clayton, Jonathan B., Robin R. Shields‐Cutler, Susan L. Hoops, Gabriel A. Al‐Ghalith, John C. M. Sha, Timothy J. Johnson, and Dan Knights. “Bacterial Community Structure and Function Distinguish Gut Sites in Captive Red‐shanked Doucs ( Pygathrix Nemaeus ).” American Journal of Primatology 81, no. 10–11 (October 2019): e22977. https://doi.org/10.1002/ajp.22977.
Guevara, Elaine E., Lydia K. Greene, Marina B. Blanco, Casey Farmer, Jeannin Ranaivonasy, Joelisoa Ratsirarson, Karine L. Mahefarisoa, et al. “Molecular Adaptation to Folivory and the Conservation Implications for Madagascar’s Lemurs.” Frontiers in Ecology and Evolution 9 (October 6, 2021): 736741. https://doi.org/10.3389/fevo.2021.736741.
Guevara, Elaine E., Timothy H. Webster, Richard R. Lawler, Brenda J. Bradley, Lydia K. Greene, Jeannin Ranaivonasy, Joelisoa Ratsirarson, et al. “Comparative Genomic Analysis of Sifakas ( Propithecus ) Reveals Selection for Folivory and High Heterozygosity despite Endangered Status.” Science Advances 7, no. 17 (April 23, 2021): eabd2274. https://doi.org/10.1126/sciadv.abd2274.
Hume, Ian D. Digestive Physiology and Nutrition of Marsupials. Monographs on Marsupial Biology. Cambridge [Cambridgeshire] ; New York: Cambridge University Press, 1982.
Liu, Jiang, Xiao-ping Wang, Soochin Cho, Burton K. Lim, David M. Irwin, Oliver A. Ryder, Ya-ping Zhang, and Li Yu. “Evolutionary and Functional Novelty of Pancreatic Ribonuclease: A Study of Musteloidea (Order Carnivora).” Scientific Reports 4, no. 1 (May 27, 2014): 5070. https://doi.org/10.1038/srep05070.
Liu, Rui, Katherine Amato, Rong Hou, Andres Gomez, Derek W. Dunn, Jun Zhang, Paul A. Garber, et al. “Specialized Digestive Adaptations within the Hindgut of a Colobine Monkey.” The Innovation 3, no. 2 (March 2022): 100207. https://doi.org/10.1016/j.xinn.2022.100207.
“Origins of New Genes and Pseudogenes | Learn Science at Scitable.” Accessed January 30, 2024. http://www.nature.com/scitable/topic...seudogenes-835.
Pozzi, Luca, Jason A. Hodgson, Andrew S. Burrell, Kirstin N. Sterner, Ryan L. Raaum, and Todd R. Disotell. “Primate Phylogenetic Relationships and Divergence Dates Inferred from Complete Mitochondrial Genomes.” Molecular Phylogenetics and Evolution 75 (June 2014): 165–83. https://doi.org/10.1016/j.ympev.2014.02.023.
Schienman, John E., Robert A. Holt, Marcy R. Auerbach, and Caro-Beth Stewart. “Duplication and Divergence of 2 Distinct Pancreatic Ribonuclease Genes in Leaf-Eating African and Asian Colobine Monkeys.” Molecular Biology and Evolution 23, no. 8 (August 1, 2006): 1465–79. https://doi.org/10.1093/molbev/msl025.
Wang, XiaoYan, NengZhi Li, Li Yu, Hui Zhao, and YaPing Zhang. “Duplication and Functional Diversification of Pancreatic Ribonuclease (RNASE1) Gene.” Chinese Science Bulletin 55, no. 1 (January 2010): 2–6. https://doi.org/10.1007/s11434-009-0717-y.
Xu, Huihui, Yang Liu, Fanxing Meng, Beibei He, Naijian Han, Gang Li, Stephen J. Rossiter, and Shuyi Zhang. “Multiple Bursts of Pancreatic Ribonuclease Gene Duplication in Insect-Eating Bats.” Gene 526, no. 2 (September 2013): 112–17. https://doi.org/10.1016/j.gene.2013.04.035.
Yu, L., and Y.-p. Zhang. “The Unusual Adaptive Expansion of Pancreatic Ribonuclease Gene in Carnivora.” Molecular Biology and Evolution 23, no. 12 (August 30, 2006): 2326–35. https://doi.org/10.1093/molbev/msl101.
Yu, Li, Xiao-yan Wang, Wei Jin, Peng-tao Luan, Nelson Ting, and Ya-ping Zhang. “Adaptive Evolution of Digestive RNASE1 Genes in Leaf-Eating Monkeys Revisited: New Insights from Ten Additional Colobines.” Molecular Biology and Evolution 27, no. 1 (January 2010): 121–31. https://doi.org/10.1093/molbev/msp216.
Zhang, J. “Parallel Functional Changes in the Digestive RNases of Ruminants and Colobines by Divergent Amino Acid Substitutions.” Molecular Biology and Evolution 20, no. 8 (April 25, 2003): 1310–17. https://doi.org/10.1093/molbev/msg143.
Zhang, Jianzhi. “Phylogenetic Evidence for Parallel Adaptive Origins of Digestive RNases in Asian and African Leaf Monkeys: A Response to Xu et al. (2009).” Molecular Phylogenetics and Evolution 53, no. 2 (November 2009): 608–9. https://doi.org/10.1016/j.ympev.2009.07.003.
Zhang, Jianzhi, Ya-ping Zhang, and Helene F. Rosenberg. “Adaptive Evolution of a Duplicated Pancreatic Ribonuclease Gene in a Leaf-Eating Monkey.” Nature Genetics 30, no. 4 (April 2002): 411–15. https://doi.org/10.1038/ng852.