29.4: Skeletal Muscle Regulates Metabolism
- Page ID
- 53876
Josep M. Argilés, Nefertiti Campos, José M. Lopez-Pedrosa, Ricardo Rueda, Leocadio Rodriguez-Mañas. Journal of the American Medical Directors Association,
Volume 17, Issue 9, 2016, Pages 789-796,ISSN 1525-8610,https://doi.org/10.1016/j.jamda.2016.04.019.
Under a Creative Commons license
Abstract
Skeletal muscle is recognized as vital to physical movement, posture, and breathing. In a less known but critically important role, muscle influences energy and protein metabolism throughout the body. Muscle is a primary site for glucose uptake and storage, and it is also a reservoir of amino acids stored as protein. Amino acids are released when supplies are needed elsewhere in the body. These conditions occur with acute and chronic diseases, which decrease dietary intake while increasing metabolic needs. Such metabolic shifts lead to the muscle loss associated with sarcopenia and cachexia, resulting in a variety of adverse health and economic consequences. With loss of skeletal muscle, protein and energy availability is lowered throughout the body. Muscle loss is associated with delayed recovery from illness, slowed wound healing, reduced resting metabolic rate, physical disability, poorer quality of life, and higher health care costs. These adverse effects can be combatted with exercise and nutrition. Studies suggest dietary protein and leucine or its metabolite β-hydroxy β-methylbutyrate (HMB) can improve muscle function, in turn improving functional performance. Considerable evidence shows that use of high-protein oral nutritional supplements (ONS) can help maintain and rebuild muscle mass and strength. We review muscle structure, function, and role in energy and protein balance. We discuss how disease- and age-related malnutrition hamper muscle accretion, ultimately causing whole-body deterioration. Finally, we describe how specialized nutrition and exercise can restore muscle mass, strength, and function, and ultimately reverse the negative health and economic outcomes associated with muscle loss.
Keywords
Muscle
glucose
amino acid
sarcopenia
HMB
ONS
Skeletal muscle is integral to physical movement, posture, and vital actions, such as chewing, swallowing, and breathing. Skeletal muscle also serves as a regulator of interorgan crosstalk for energy and protein metabolism throughout the body, a less recognized but critically important role. As such, skeletal muscle is a key site for glucose uptake and storage.3 Skeletal muscle is likewise a reservoir of amino acids that can support protein synthesis or energy production elsewhere in the body when other sources are depleted.4
This review of muscle metabolism describes how amino acids stored as protein in muscle can be broken down through proteolysis for ultimate use in energy production. Such breakdown occurs when energy demands are high (as with stress-induced hypermetabolism), or when supplies are low (as in severe starvation or longer-term protein energy malnutrition). Both of these states can be hallmarks of many diseases, either directly as a result of disease-related dysregulation of metabolism (such as in the extreme case of cancer-cachexia) or, more subtly, as a result of the general illness-associated loss of appetite. Muscle is therefore crucially important during illness, both for its role in balancing the metabolic needs of other organs and for its reserves of protein for use in energy production. Yet, during illness, the maintenance of muscle mass through exercise and nutrition are often overlooked or difficult to address, and muscle atrophy develops. Even more subtle is aging-related muscle loss, which can dramatically increase morbidity and mortality of otherwise survivable illnesses in the aged. This review also illustrates the consequences of muscle atrophy in aging and illness and proposes steps to combat these challenges.
Muscle Basics
Muscle Structure and Classification
Skeletal muscle comprises the fibrillar proteins myosin (a thick filament) and actin (a thin filament) that interact to cause muscle contraction, a process requiring energy in the form of adenosine triphosphate (ATP). Different muscle types have been classified according to histochemical features, structural protein composition, and major metabolic properties.5, 6 Most commonly, skeletal muscles are referred to as either “slow” or “fast” to reflect speeds of contraction, or the shortening of myosin heavy chain (MHC) protein.6 The velocity of this shortening is dependent on the MHC isoform present; “fast” fiber isoforms MHCIIa and IIb demonstrate a higher shortening velocity than their “slow” fiber MHCI counterparts.6, 7 Classic histochemical staining methods also classify muscle as type I (slow) and type II (fast) based on the myosin ATPase enzyme forms revealed. Recently, these types have been further distinguished based on histology (types I, IC, IIC, IIAC, IIA, IIAB, and IIB).6
Muscle Metabolism and Interorgan Crosstalk
Glucose regulation is central to energy balance both within muscle fibers and throughout the body. In the cytoplasm of most cells, glucose undergoes glycolysis to produce the substrate for ATP generation. Muscle fibers are also characterized on the basis of the speed and manner in which they metabolize glucose. The terms “fast” and “slow” can indicate the type of glucose metabolism occurring within the fiber. Slow muscles, which use aerobic metabolism, contain a high density of capillaries and oxidative enzymes that allow a greater resistance to fatigue.7 Fast muscles, which depend on anaerobic metabolism, or glycolysis, can quickly generate ATP and therefore contract more readily. Fast muscles also fatigue sooner than slow fibers, as the conversion of glucose to pyruvate generates less ATP than can be generated by using the rest of central metabolism, ultimately generating CO2.
Muscle has the ability to store glucose in the form of glycogen, which facilitates the rapid initiation of energy production for contraction even when glucose is not readily available from the diet. This unique capacity, shared also by the liver and kidneys, makes skeletal muscle an important metabolic organ that helps all organs have access to essential energy substrates during fasting. Furthermore, the amino acids stored in muscle as protein can be broken down as a last resort during times of starvation or extreme energy shortfalls.4 Patterns of glucose utilization throughout the body as a whole reflect feeding status (Figure 1; Table 1). Based on a classic study of the fed state (measurement within 3 hours of eating), researchers estimated that 25% to 35% of an ingested carbohydrate load was quickly extracted from circulation and stored by the liver.3 Of the remaining glucose, approximately 40% was disposed in the muscle and 10% in the kidney.3 The brain used 15% to 20% of post-meal glucose.3
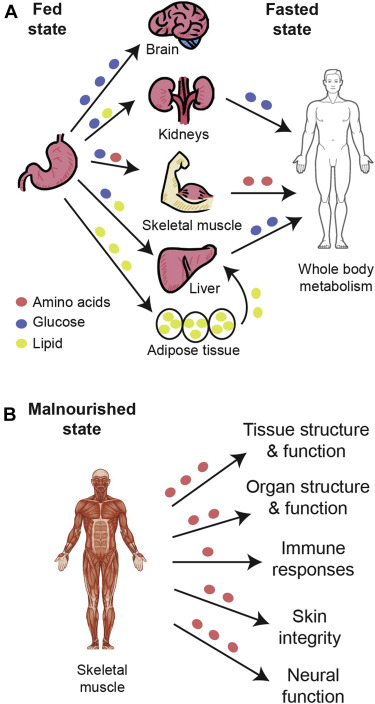
Fig. 1. Glucose metabolism in fed, fasted and malnourished states. A, Glucose, lipids, and amino acids from the diet circulate during the fed state for use or storage in body organs. In the fasted state, glucose is released from the muscles, kidneys, and liver for whole-body metabolism, along with lipids from adipose tissue and amino acids from the muscle. B, When glucose stores have been depleted, amino acids are provided by the muscles to support crucial bodily functions.
Table 1. Glucose Metabolism in Fasted and Fed States
Fed State | Fasted State |
---|---|
Diet-sourced glucose (exogenous glucose) is absorbed from the intestine to circulate in blood; glucose serves as an energy source in cells throughout the body. Cytoplasmic glucose undergoes glycolysis, in turn producing ATP. | Little or no blood glucose from dietary sources; alternative energy sources are needed for function of tissues body-wide. |
Glucose is primarily taken up by muscle and liver, where it can be used for energy or stored as glycogen (Glycogen synthesis). | Glycogen stored in liver, kidney, and muscle is broken down to provide glucose as energy source (Glycogenolysis). Muscle uses glycogen-sourced glucose internally; liver and kidney can supply glucose to circulation. |
Gluconeogenic substrates are stored in various organs (eg, pyruvate in liver, glycerol in fat, and amino acids in muscle). | Endogenous generation of glucose from noncarbohydrate carbon substrates, such as pyruvate, lactate, glycerol, and glucogenic amino acids (Gluconeogenesis); occurs primarily in the liver and muscle, and to a lesser extent in the kidney. |
In the fasted state (after 14 to 16 h without eating), the liver provides approximately 80% of glucose that is released into circulation. About half of this glucose comes from the breakdown of stored glycogen, and the rest from the metabolism of sources other than carbohydrate or glycogen, including certain amino acids, through a process known as gluconeogenesis.8 Interactions between muscle and liver are largely responsible for regulating carbohydrate metabolism and for achieving energy balance in normal fed and fasted states; the kidneys play a role similar to that of the liver, but to a lesser extent.3, 8 In addition, muscle tissue stores amino acids as protein, and adipose tissue serves as a depot of glycerol and fatty acids. As needed, amino acids and fatty acids can be metabolized to form acetyl coenzyme A for the tricarboxylic acid (TCA) cycle.
As glycogen stores become depleted, increasingly more glucose is produced by gluconeogenesis. Gluconeogenesis provides 70% of glucose released into the body 24 hours after eating, and 90% by 48 hours.8 As fasting is prolonged, the kidneys contribute increasingly higher amounts of glucose from gluconeogenesis.
Ultimately, amino acids stored in skeletal muscle are metabolized when the need for gluconeogenesis substrate is greatest. Skeletal muscle houses nearly 75% of all protein in the body and constitutes an important contributor to gluconeogenesis in states of drastic depletion. Maintenance of muscle protein content depends on the balance between protein synthesis and degradation.5 Under normal conditions, muscle protein mass gains during the fed state balance losses during the fasted state.4 However, under severe metabolic stress generated by serious illness or injury, muscle protein can become depleted by catabolism, and this can lead to harmful functional limitations.
Skeletal muscle proteolysis can provide amino acid substrates for glucose and glycogen formation, notably glutamine and alanine. Alanine is released into circulation and reaches the liver, where it serves as an excellent substrate for gluconeogenesis. Glutamine also has a beneficial role in this process: the carbon skeleton of glutamine is a gluconeogenic precursor that can regulate gluconeogenesis independently of the insulin/glucagon ratio. Therefore, glutamine supplementation may also enhance glycogen synthesis and increase muscle glycogen stores even when insulin levels are low or when insulin resistance is present.9
In summary, dietary glucose is supplied by meals, and glucose is stored as glycogen in liver, kidney, and muscle for metabolic energy functions, as needed (Table 1). At times when glucose supplies are not sufficient to meet energy needs, breakdown of glycogen (glycogenolysis) occurs. When stored glucose products are no longer available, energy is released by breakdown of substrates other than glucose. In this review of muscle metabolism, we emphasize that amino acids stored as protein in muscle can be broken down by way of gluconeogenesis, ultimately entering the TCA cycle for energy production. Such breakdown occurs when energy demands are high, as with stress-induced hypermetabolism of disease, or when supplies are low, as in severe starvation or disease-associated loss of appetite. Such use can become problematic in that it reduces skeletal muscle mass and produces waste nitrogen, which requires further energy to sequester and secrete. Prolonged reliance on these processes can accelerate existing health problems and must be addressed by the health care provider.
Muscle Plasticity: Changes in Muscle Mass, Strength, and Function
Skeletal muscle is remarkably plastic. It changes continuously in response to calorie and nutrient intake, illness, and physical stress. Changes in adult skeletal muscle also may occur as fiber-type switching, which is influenced by changes in physical activity, loading, nerve stimulation, or hormone and cytokine levels.7, 10, 11, 12
Mechanisms of Muscle Growth and Strength Increase
Muscle adapts positively to demands placed on it, such as the increased contractile activity associated with endurance training or the increased loading attributable to strength training. This tremendous plasticity is evident as muscle tissue accretion, specific changes within muscle, and muscle tissue breakdown.1 Muscle growth, or hypertrophy, occurs when protein synthesis within the muscles outpaces protein degradation. This process can be positively regulated by mammalian target of rapamycin (mTOR) signaling induced by insulin after calorie ingestion, by hormones such as testosterone, and by exercise.10
Mechanisms of Muscle Loss in Aging, Inactivity, Sickness, and Frailty
This ability of skeletal muscle to change dynamically in response to body conditions is also manifest as changes resulting from injury, illness, or aging. When the metabolic demands placed on muscle outweigh the protein synthesis that occurs from dietary intake and after exercise, muscle mass is lost, metabolic storage products are depleted, and muscle fiber balance changes.
Aging may lead to a loss of muscle mass resulting from both the shrinking of muscle fibers (atrophy) and the elimination of fibers altogether (Figure 2).6 This condition is known as primary sarcopenia, the age-related loss of muscle mass and function. Although both fiber types I and II lose mass, aging causes preferential atrophy of type II fibers; the net change is thus from type II to type I fibers, or from fast to slow muscle fibers.6, 13 Because fast muscle fibers mobilize ATP and create tension more readily than slow fibers, this shift can leave older adults without the energy to perform daily tasks.14 This shift to type I slow fibers leads to a corresponding increase in their characteristic oxidative metabolism relative to the glycolytic metabolism that occurs in type II fast fibers. Exacerbating the problems caused by muscle degradation in aging, it is possible that type I oxidative fibers normally experience higher protein turnover (i. protein synthesis and degradation), are less able to grow in size, and have different responses to insufficient nutrient intake, although these fiber-type differences remain poorly understood.7, 15, 16
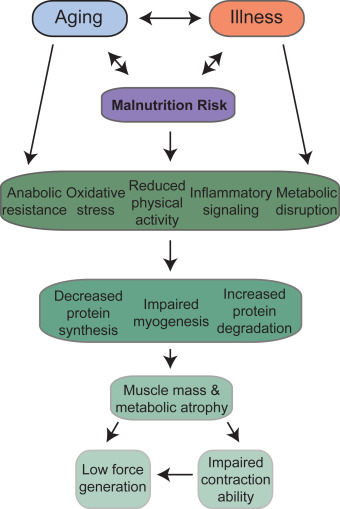
Fig. 2. Effects of aging and illness on muscle mass.
Beyond aging, muscle wasting is associated with many pathological states and chronic diseases, such as malnutrition, cancer, chronic kidney disease, chronic obstructive pulmonary disease, burns, muscular dystrophies, acquired immunodeficiency syndrome, sepsis, and immune disorders, and forced immobilization and bed rest are devastating to patients who are already challenged by these factors (Figure 2).14, 17 Most of these pathological conditions are associated with variable degrees of local and/or systemic chronic inflammation, which plays a crucial role in the onset of muscle atrophy. Loss of muscle mass is frequently associated with increased production of proinflammatory cytokines. Systemic inflammation is associated with reduced rates of protein synthesis paralleled by enhanced protein breakdown, both accounting for the loss of muscle mass. The effects exerted by proinflammatory cytokines on muscle mass are partially mediated by activating the transcription factor nuclear factor κB (NF-κB).18 The transcriptional activity is regulated by the phosphorylation and consequent degradation of the inhibitor Iκ-Bα, allowing the positive regulation of muscle RING-finger protein-1 (MuRF1) and other atrophy-related genes. Proinflammatory cytokines act on muscle protein metabolism not only by activating catabolic pathways, but also by downregulating the anabolic pathways.19 Elevated tumor necrosis factor-alpha (TNF-α) and interleukin-1 (IL-1) lead to inhibition of the Akt/mTOR signal transduction pathway and a subsequent reduction in protein synthesis. The inflammatory process that takes place during trauma or fractures is controlled and finely regulated. In the short term, it can facilitate complete and efficient reconstruction of muscle fibers through the stimulation of myogenesis. However, chronic inflammation can be deleterious, driving uncontrolled muscle atrophy and affecting contraction ability. Balance between pro- and anti-inflammatory cytokines is well known to be important in regulating physiological muscle protein turnover and myogenesis, and evidence suggesting that inflammation can impair force generation in muscles is also growing.20, 21
As inflammation accelerates muscle catabolism, resting energy expenditure increases and amino acids are released from muscles to serve as substrates for gluconeogenesis in liver and elsewhere in the body (Table 2).22 The efficiency of energy production is low when amino acids are used to generate energy, so muscle is at further risk for breakdown to meet needs.23 In addition, the liver changes metabolic priorities, using amino acids to produce acute phase reactant proteins instead of normal proteins, such as serum albumin, and to support gluconeogenesis. This process continues until the cause of stress has subsided. Thus, when the dietary proteins supplied are inadequate to meet needs, muscle protein is broken down to supply amino acids throughout the body. This reaction releases waste nitrogen, which requires further energy to convert to urea, thereby exacerbating the problem of the energy shortfall.24
Table 2. Major Molecular Pathways Influencing Muscle Accretion
Effector | Mediator | Major Pathway(s) | Consequence |
---|---|---|---|
Mammalian target of rapamycin (mTOR) | +Induced by BCAAs, HMB | Interacts with protein translation machinery to facilitate initiation and elongation | mTOR stimulation by a number of pathways increases protein synthesis |
Insulinlike growth factor (IGF1) | +Stimulated by meal-induced insulin | IGF1R → PI3K → AKT → mTOR | Reduced IGF1 from decreased eating and/or exercise leads to reduced protein synthesis and to muscle wasting |
+Stimulated by exercise | |||
Myostatin/Activin | +Produced by skeletal muscle | Activin receptors (ACTRIIA/B) → Smad2/3 –I mTOR | Myostatins negatively regulate protein synthesis |
–Inhibited by Follistatin | ACTRIIA/B → FoxO → UPS | ||
Inflammatory cytokines (TNFα, IL-1) | +Upregulated by illness, injury | Cytokine receptors → NFKB, p38, JAK, Caspases, E3 ligases | Inflammation leads to apoptosis or autophagy-mediated muscle cell loss |
–Inhibited by exercise | FoxO transcription factors → MAFBX; MURF1 → UPS (ubiquitin-proteasome system) | ||
Vitamin D | +Levels are increased by diet and sunlight | Vitamin D receptor → gene expression or repression in myogenic cells | Vitamin D positively influences muscle growth |
AKT, protein kinase B; BCAA, branched-chain amino acid; FoxO, forkhead box protein O; HMB, β-Hydroxy β-Methylbutyrate; IGF1R, insulin-like growth factor 1 receptor; JAK, janus kinase; MURF1, muscle RING-finger protein-1 p38, mitogen-activated protein kinase; PI3K, phosphatidylinositide 3-kinase; UPS, ubiquitin-proteasome system.
Complications Associated With Loss of Muscle
As aging and illness lead to muscle breakdown and atrophy, reduced muscle mass leaves patients without a crucial reservoir of amino acids and effector molecules, such as myokines, cytokines released by muscle, to help the body combat illness, infection, and wasting (Figure 3).23, 25, 26, 27 Therefore, muscle atrophy is associated with a wide range of harmful health effects that can be life changing, especially for older people.28, 29, 30, 31, 32, 33, 34, 35, 36 The most relevant condition associated with the presence of sarcopenia in this population is a clinical syndrome called frailty. The most accepted physiological framework explaining frailty and its consequences was proposed by Walston and Fried,37 who described a relationship between sarcopenia and energy imbalance called the “frailty cycle.” This cycle affects multiple systems, especially those susceptible to changes in hormones (mainly sexual hormones, IGF-1, and insulin) and the progressive development of a proinflammatory state.38, 39, 40 Additional biomarkers have recently been identified for roles in frailty, such as those related to endothelial dysfunction or micro RNAs central to the aging process.41, 42 Frailty can be defined as an age-associated biological syndrome characterized by a decreased biological reserve resulting from a decline in multiple physiological systems that leaves the individual at risk for developing poor outcomes (disability, death, and hospitalization) in the presence of stressors.43, 44 The prevalence of frailty in people older than 65 is approximately 10%, increases with age, and is greater in women.45
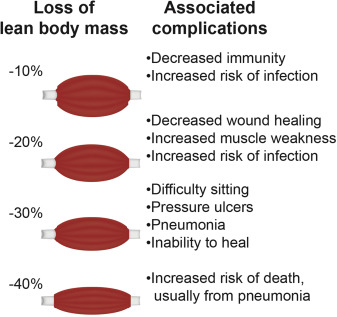
Fig. 3. Complications of lean body mass (muscle) loss.
Frailty is now a recognized clinical medical syndrome that provides a biological framework for understanding vulnerabilities resulting from aging or chronic conditions.44, 46 It is clinically important to detect frailty in those at risk of developing disability. As aging progresses, frailty increases as the prognostic factor for death and incident disability.47, 48 Frailty and its underlying sarcopenia have been shown to predict risk of death, disability, and other adverse outcomes, including muscle mass atrophy, metabolic deterioration, slowed wound or postsurgical healing, and delayed recovery from illness.32, 34, 35 Frailty and the weakness that follows muscle loss lead to higher risk of falls, fractures,30 physical disability,29 need for institutional care,29 reduced quality of life,36 and heightened mortality.29, 33 Early identification of frailty risk provides the opportunity to provide interventions and avoid or delay disability as well as enhance recovery.
Loss of muscle associated with disease, injury, disuse, or aging significantly increases the cost of health care.34, 49, 50 Results of a recent study showed that older adults (mean age=70 years) who were very frail spent (Euro) 1917 more on total health costs in an interval of 3 months than did those who were not frail.51 In the United States, the direct cost of cachexia/sarcopenia to health care was reported to be 1.5% of annual total health care expenses.50 Such costs arise from the increased rate of hospitalization, incidence of complications, lengths of stay, and likelihood of readmission.52, 53 In the face of an aging population, the importance of identifying, preventing, and treating muscle loss cannot be overstated.
Detection and Treatment of Muscle Loss
Who Is at Risk of Muscle Atrophy, and How Do We Identify It?
Screening is crucial for predicting risk, and proper, timely intervention can reduce or eliminate the ensuing muscle mass and metabolic atrophy, substantially affecting morbidity, mortality, and cost. Special attention should be paid to the main risk categories: people who are malnourished or at risk of malnutrition for any reason33, 54; frail adults, especially the very old; people who become deconditioned and lose muscle due to age- and disability-related physical inactivity35; those with diseases or conditions with inflammatory components, such as chronic heart failure,55 chronic or acute kidney disease,56 cancer,57, 58, 59 severe infection and sepsis,60 insulin resistance/diabetes,61 intensive care unit–acquired weakness,25 and wound/surgical recovery.34
Reaching an accurate diagnosis of age- or disease-related muscle atrophy is difficult, and a number of criteria have been proposed but have not yet assessed in the clinical setting.14 Nonetheless, specific criteria and measures can be used to diagnose sarcopenia or cachexia.13, 27, 62, 63 Sarcopenia can be diagnosed when a patient has muscle mass that is at least 2 SDs below the relevant population mean and also presents with a low gait (walking) speed. In addition, low muscle strength and general physical performance may be taken into consideration.14 Cachexia can be diagnosed when at least 5% of body weight is lost within 12 months in the presence of underlying illness, and 3 of the following criteria are also met: decreased muscle strength, increased fatigue, anorexia, low fat-free mass index, abnormal biochemistry, increased inflammatory markers C-reactive protein (>5.0 mg/L) or IL-6 (>4.0 pg/mL), anemia (<12 g/dL), or low serum albumin (<3.2 g/dL).
Recent research into the molecular adaptations associated with the development of or that result from muscle atrophy and metabolic depletion may lead to the identification of biomarkers and, therefore, improvements in early detection (Table 2). A variety of signaling pathways known to positively influence muscle growth (bone morphogenetic proteins, brain-derived neurotrophic factors, follistatin, and irisin), as well as those known to negatively regulate muscle growth (transforming growth factor β, myostatin, activins, and growth and differentiation factor-15) and factors associated with muscle function and dysfunction (C-terminal agrin fragment and skeletal muscle specific troponin T) may emerge as biomarkers for muscle atrophy in aging and disease.64 To date, there is no universally recognized biomarker for muscle atrophy, but recent research in the field suggests that the combination of several biomarkers may facilitate the adequate diagnosis of muscle atrophy. Identification of such biomarkers and their incorporation into validated testing instruments should allow early identification of muscle atrophy (improving prognosis, and likely reducing cost to health care systems), but may also provide exciting targets for the development of new medications.
Nutritional Strategies for Maintaining and Rebuilding Muscle
Treatment of patients at risk can prevent or delay onset of muscle atrophy, or even target rebuilding of muscle when muscle atrophy is already evident (Figure 4).65 As a first step, treatment must provide adequate energy so that muscle proteins and their constituent amino acids are spared as an energy source. In addition, high protein intake is vital to treatment of muscle atrophy or for delaying its onset.7, 66, 67, 68, 69 It should be noted that the range of protein needs can vary widely from patient to patient. Because muscle mass may decrease or remain the same (based largely on how much protein synthesis outpaces protein degradation), the most direct way to prevent muscle loss is to ensure that sufficient protein is ingested. Use of high-protein oral nutritional supplements (ONS; ≥20% of total calories as protein) may be beneficial to such patients.70
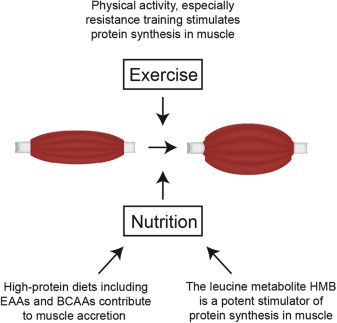
Fig. 4. Treatments for sarcopenia. It is currently recommended that patients at risk of or suffering from sarcopenia consume a diet high in protein, engage in resistance exercise, and take supplements of the leucine metabolite HMB.
By definition, the essential amino acids (EAAs) play a central role in protein nutritional status. Some amino acids play roles that are distinct from the traditional one of protein building blocks; many of these have little or nothing to do with protein synthesis, and are thus not included here. However, of central importance to the current discussion are the branched-chain amino acids (BCAAs), especially leucine.65, 71, 72 BCAAs promote protein synthesis in the muscles through a number of pathways.66 In particular, they are now known to have a key role in altering tissue response to a meal, the post-prandial response, especially in muscle, where they signal a reduction in protein breakdown and an increase in protein synthesis, resulting in net accretion of protein in muscle and helping to regulate blood amino acid levels. However, aspects of this postprandial regulation are not as robust in aged muscle, and muscle in hypercatabolic conditions, such as cancer, is challenged and its normal system is overwhelmed. In these cases, a substantial body of research suggests that significantly more of these amino acids are required in the diet to overcome resistance to protein anabolism; very high doses, such as 10 to 15 g of BCAAs, or 3 g or more of leucine per meal, have been studied to combat muscle loss in the elderly,44 although this may be a result of improved protein synthesis that does not lead to muscle mass accretion.73, 74, 75
This resistance to the normal of BCAAs in muscle protein homeostasis has prompted studies into leucine's mechanism of action. These have identified the leucine metabolite β-hydroxy β-methylbutyrate (HMB) as a potent stimulator of protein synthesis as well as an inhibitor of protein breakdown in the extreme case of cachexia.65, 72, 76, 77, 78, 79, 80, 81, 82, 83, 84 A growing body of evidence suggests HMB may help slow, or even reverse, the muscle loss experienced in sarcopenia and improve measures of muscle strength.44, 65, 72, 76, 77, 78, 79, 80, 81, 82, 83, 84 However, dietary leucine does not provide a large amount of HMB: only a small portion, as little as 5%, of catabolized leucine is metabolized into HMB.85 Thus, although dietary leucine itself can lead to a modest stimulation of protein synthesis by producing a small amount of HMB, direct ingestion of HMB more potently affects such signaling, resulting in demonstrable muscle mass accretion.71, 80 Indeed, a vast number of studies have found that supplementation of HMB to the diet may reverse some of the muscle loss seen in sarcopenia and in hypercatabolic disease.65, 72, 83, 86, 87 The overall treatment of muscle atrophy should include dietary supplementation with HMB, although the optimal dosage for each condition is still under investigation.68
In addition to dietary protein, EAAs/BCAAs including leucine, the leucine metabolite HMB, a number of other dietary or supplemental components have been explored for their ability to positively influence muscle mass during sarcopenia. These include creatine monohydrate, a variety of antioxidants, ornithine α-ketoglutarate, omega-3 fatty acids, ursolic acid, and nitrates.68, 88, 89, 90 Given the length of this list, its growing nature, and the difficulty many aged individuals experience ingesting proper calories and nutrients, additional studies will be needed to determine which components are most beneficial to maintaining muscle mass, as well as their optimal doses and administration routes both in isolation and in combination.
Physical Activity Is Also Key
Nutrition is important and can counteract metabolic alterations induced during periods of significant stress and inflammation; however, sufficient exogenous provisions of protein and energy substrates alone cannot completely eliminate or reverse the deteriorations associated with aging or the deleterious impact inadequate control and regulation of inflammation have on muscle.23, 66, 69 Protein synthesis occurs in muscle fibers following their contraction,91 and physical activity has been shown to induce a number of anabolic signaling pathways.92 Physical activity can likewise reduce degradation of muscle protein.93, 94 Even more, a lack of physical activity increases the resistance of muscle to anabolism, particularly the synthesis of proteins from amino acids.95 An exercise component to muscle atrophy treatment is therefore highly recommended, and exercise also may prevent the onset of sarcopenia later in life, possibly by increasing the presence of type I fibers that are less susceptible to degradation during sarcopenia.7, 25, 66, 89 Although aerobic and other types of exercise are all preferable to a lack of physical activity, resistance exercise in particular has been shown repeatedly to improve rates of protein synthesis and reverse muscle loss.88, 89, 94 This may be attributable to differential effects on muscle fiber types.7 It is therefore recommended that patients with muscle atrophy or at risk of developing muscle atrophy engage a regular exercise program containing both aerobic and anaerobic components, and the importance of appropriate resistance training cannot be overstated. Although this must be tailored to the individual's current physical status, it should also periodically be reviewed and increased to maximize its impact.
Although resistance exercise and general physical activity are important to the stimulation of protein synthesis from amino acids in the diet, some aged and ill individuals experiencing extensive muscle atrophy are likely to have difficulty engaging in physical activities because of low energy and other medical complications. Nutrition and some supplements can be used to bolster results of exercise, both preventively and during sarcopenia. For example, bioactive substances known as nutraceuticals that mimic the molecular effects of exercise can induce signaling pathways that are thought to support or even underlie exercise's effects on health and muscle mass accretion. Found in a variety of foods, including some common fruits, green tea, and even red wine, these compounds can be isolated and added to nutritional supplements used in the treatment of muscle atrophy.44
Summary and Conclusions
The classic physical functions of skeletal muscle are well known, but skeletal muscle is increasingly recognized as a one of the key regulators of energy and protein metabolism by way of metabolic crosstalk between body organs. Skeletal muscle is the primary site for glucose uptake and storage, and it is likewise a reservoir of amino acids that sustain protein synthesis in all other body sites. When dietary glucose intake decreases or metabolic needs increase, stored glucose is mobilized from liver, while energy is released from fat depots. When these energy supplies are depleted, the muscle reservoir of amino acids stores is tapped, and muscle proteins are broken down to provide amino acids for gluconeogenesis, thereby supplying energy to other parts of the body.
Undernutrition and resultant muscle loss (muscle atrophy), as associated with aging and disease, can lead to adverse health and economic consequences. Conditions and diseases that lower dietary intake and increase nutrient needs are associated with catabolism of skeletal muscle, which in turn limits availability of protein and energy throughout the body. Loss of muscle mass, strength, and function has adverse consequences: slowed wound healing and recovery from illness, physical disability (due both to overall reduction of muscle status), as well as selective losses in type I fibers, which are essential for balance recovery (and thus fall prevention), poorer quality of life, and higher health care costs.
Nutrition and exercise are key to growth and maintenance of muscle promoting overall health, well-being, and recovery from disease. A wealth of research underscores the importance of a few key dietary components: protein (EAAs/BCAAs in particular), and the leucine metabolite HMB. Others will very likely be added to this list as our knowledge base grows. In addition, physical activity, especially resistance strength training, is essential to the treatment of muscle atrophy. Others will very likely be added to this list as our knowledge base grows. Considerable evidence shows that ONS and enteral feeding formulations can help maintain and rebuild muscle mass and strength. Further studies are needed to show support for functional outcomes, such as ability to perform activities of daily living and maintain or restore independence.
Acknowledgments
The authors thank Jeffrey H. Baxter and Abby Sauer from ANR&D for their critical review of this article, as well as Cecilia Hofmann, PhD, and Hilary North Scheler, PhD (C Hofmann & Associates, Western Springs, IL), for valuable assistance with efficient compilation of the medical literature and with editing this English-language review article.
References
- 1
- J.A. Chromiak, J. Antonio
Skeletal muscle plasticity
J. Antonio, D. Kalman, J. Stout (Eds.), Essentials of Sports Nutrition and Supplements, Humana Press, Totowa, NJ (2008)
- 2
- H. Shiozu, M. Higashijima, T. Koga
Association of sarcopenia with swallowing problems, related to nutrition and activities of daily living of elderly individuals
J Phys Ther Sci, 27 (2015), pp. 393-396
- 3
- C. Meyer, J.M. Dostou, S.L. Welle, J.E. Gerich
Role of human liver, kidney, and skeletal muscle in postprandial glucose homeostasis
Am J Physiol Endocrinol Metab, 282 (2002), pp. E419-E427
- 4
- R.R. Wolfe
The underappreciated role of muscle in health and disease
Am J Clin Nutr, 84 (2006), pp. 475-482
- 5
- W.R. Frontera, J. Ochala
Skeletal muscle: a brief review of structure and function
Calcif Tissue Int, 96 (2015), pp. 183-195
- 6
- W. Scott, J. Stevens, S.A. Binder-Macleod
Human skeletal muscle fiber type classifications
Phys Ther, 81 (2001), pp. 1810-1816
- 7
- A. Matsakas, K. Patel
Skeletal muscle fibre plasticity in response to selected environmental and physiological stimuli
Histol Histopathol, 24 (2009), pp. 611-629
- 8
- M. Shrayyef, J. Gerich
Normal glucose homeostasis
L. Poretsky (Ed.), Principles of Diabetes Mellitus (2nd ed), Springer, New York, NY, US (2010)
- 9
- G. Perriello, N. Nurjhan, M. Stumvoll, et al.
Regulation of gluconeogenesis by glutamine in normal postabsorptive humans
Am J Physiol, 272 (1997), pp. E437-E445
- 10
- S. Schiaffino, C. Reggiani
Fiber types in mammalian skeletal muscles
Physiol Rev, 91 (2011), pp. 1447-1531
- 11
- T. Eken, K. Gundersen
Electrical stimulation resembling normal motor-unit activity: effects on denervated fast and slow rat muscles
J Physiol, 402 (1988), pp. 651-669
- 12
- D. Pette
The adaptive potential of skeletal muscle fibers
Can J Appl Physiol, 27 (2002), pp. 423-448
- 13
- S. von Haehling, J.E. Morley, S.D. Anker
From muscle wasting to sarcopenia and myopenia: update 2012
J Cachexia Sarcopenia Muscle, 3 (2012), pp. 213-217
- 14
- M.J. Tisdale
Catabolic mediators of cancer cachexia
Curr Opin Support Palliat Care, 2 (2008), pp. 256-261
- 15
- B. Mittendorfer, J.L. Andersen, P. Plomgaard, et al.
Protein synthesis rates in human muscles: Neither anatomical location nor fibre-type composition are major determinants
J Physiol, 563 (2005), pp. 203-211
- 16
- C.A. Goodman, J.A. Kotecki, B.L. Jacobs, T.A. Hornberger
Muscle fiber type-dependent differences in the regulation of protein synthesis
PLoS One, 7 (2012), p. e37890
- 17
- S. Ventadour, D. Attaix
Mechanisms of skeletal muscle atrophy
Curr Opin Rheumatol, 18 (2006), pp. 631-635
- 18
- H. Li, S. Malhotra, A. Kumar
Nuclear factor-kappa B signaling in skeletal muscle atrophy
J Mol Med (Berl), 86 (2008), pp. 1113-1126
- 19
- H.L. Eley, M.J. Tisdale
Skeletal muscle atrophy, a link between depression of protein synthesis and increase in degradation
J Biol Chem, 282 (2007), pp. 7087-7097
- 20
- D. Costamagna, P. Costelli, M. Sampaolesi, F. Penna
Role of inflammation in muscle homeostasis and myogenesis
Mediators Inflamm, 2015 (2015), p. 805172
- 21
- R.C. Langen, A.M. Schols, M.C. Kelders, et al.
Inflammatory cytokines inhibit myogenic differentiation through activation of nuclear factor-kappaB
FASEB J, 15 (2001), pp. 1169-1180
- 22
- M. Winkler, A. Malone
Medical nutrition therapy for metabolic stress: Sepsis, trauma, burns, and surgery
K. Mahon, J. Raymond, S. Escott-Stump (Eds.), Krause's Food and the Nutrition Care Process, Elsevier, St Louis, MO (2012)
- 23
- M. Fischer, A. JeVenn, P. Hipskind
Evaluation of muscle and fat loss as diagnostic criteria for malnutrition
Nutr Clin Pract, 30 (2015), pp. 239-248
- 24
- DL Nelson, MM Cox
Lehninger Principles of Biochemistry
(6th ed), W. H. Freeman & Co, New York, NY (2013), pp. 884-900
- 25
- D.K. Heyland, R.D. Stapleton, M. Mourtzakis, et al.
Combining nutrition and exercise to optimize survival and recovery from critical illness: Conceptual and methodological issues
Clin Nutr (2015), 10.1016/j.clnu.2015.07.003
- 26
- E. Curtis, A. Litwic, C. Cooper, E. Dennison
Determinants of muscle and bone aging
J Cell Physiol, 230 (2015), pp. 2618-2625
- 27
- A.J. Cruz-Jentoft, J.P. Baeyens, J.M. Bauer, et al.
Sarcopenia: European consensus on definition and diagnosis: Report of the European working group on sarcopenia in older people
Age Ageing, 39 (2010), pp. 412-423
- 28
- B.C. Clark, T.M. Manini
Functional consequences of sarcopenia and dynapenia in the elderly
Curr Opin Clin Nutr Metab Care, 13 (2010), pp. 271-276
- 29
- V. Hirani, F. Blyth, V. Naganathan, et al.
Sarcopenia is associated with incident disability, institutionalization, and mortality in community-dwelling older men: The Concord Health and Ageing in Men Project
J Am Med Dir Assoc, 16 (2015), pp. 607-613
- 30
- D. Scott, R.M. Daly, K.M. Sanders, P.R. Ebeling
Fall and fracture risk in sarcopenia and dynapenia with and without obesity: The role of lifestyle interventions
Curr Osteoporos Rep, 13 (2015), pp. 235-244
- 31
- S. Yu, K. Umapathysivam, R. Visvanathan
Sarcopenia in older people
Int J Evid Based Healthc, 12 (2014), pp. 227-243
- 32
- R.H. Demling
Nutrition, anabolism, and the wound healing process: An overview
Eplasty, 9 (2009), p. e9
- 33
- A.P. Cerri, G. Bellelli, A. Mazzone, et al.
Sarcopenia and malnutrition in acutely ill hospitalized elderly: Prevalence and outcomes
Clin Nutr, 34 (2015), pp. 745-751
- 34
- J. Friedman, A. Lussiez, J. Sullivan, et al.
Implications of sarcopenia in major surgery
Nutr Clin Pract, 30 (2015), pp. 175-179
- 35
- B.T. Wall, M.L. Dirks, L.J. van Loon
Skeletal muscle atrophy during short-term disuse: Implications for age-related sarcopenia
Ageing Res Rev, 12 (2013), pp. 898-906
- 36
- R. Rizzoli, J.Y. Reginster, J.F. Arnal, et al.
Quality of life in sarcopenia and frailty
Calcif Tissue Int, 93 (2013), pp. 101-120
- 37
- J. Walston, L.P. Fried
Frailty and the older man
Med Clin North Am, 83 (1999), pp. 1173-1194
- 38
- L. Carcaillon, F.J. Garcia-Garcia, J.A. Tresguerres, et al.
Higher levels of endogenous estradiol are associated with frailty in postmenopausal women from the Toledo study for healthy aging
J Clin Endocrinol Metab, 97 (2012), pp. 2898-2906
- 39
- B.W. Penninx, S.B. Kritchevsky, A.B. Newman, et al.
Inflammatory markers and incident mobility limitation in the elderly
J Am Geriatr Soc, 52 (2004), pp. 1105-1113
- 40
- J. Walston, M.A. McBurnie, A. Newman, et al.
Frailty and activation of the inflammation and coagulation systems with and without clinical comorbidities: Results from the Cardiovascular Health Study
Arch Intern Med, 162 (2002), pp. 2333-2341
- 41
- C. Alonso-Bouzon, L. Carcaillon, F.J. Garcia-Garcia, et al.
Association between endothelial dysfunction and frailty: The Toledo Study for Healthy Aging
Age (Dordr), 36 (2014), pp. 495-505
- 42
- L. Rodriguez Manas
Determinants of frailty and longevity: Are they the same ones?
Nestle Nutr Inst Workshop Ser, 83 (2015), pp. 29-39
- 43
- L. Rodriguez-Manas, C. Feart, G. Mann, et al.
Searching for an operational definition of frailty: A Delphi method based consensus statement: The frailty operative definition-consensus conference project
J Gerontol A Biol Sci Med Sci, 68 (2013), pp. 62-67
- 44
- A. Clegg, J. Young, S. Iliffe, et al.
Frailty in elderly people
Lancet, 381 (2013), pp. 752-762
- 45
- R.M. Collard, H. Boter, R.A. Schoevers, R.C. Oude Voshaar
Prevalence of frailty in community-dwelling older persons: A systematic review
J Am Geriatr Soc, 60 (2012), pp. 1487-1492
- 46
- L. Rodriguez-Manas, L.P. Fried
Frailty in the clinical scenario
Lancet, 385 (2015), pp. e7-e9
- 47
- P. Abizanda, L. Romero, P.M. Sanchez-Jurado, et al.
Age, frailty, disability, institutionalization, multimorbidity or comorbidity. Which are the main targets in older adults?
J Nutr Health Aging, 18 (2014), pp. 622-627
- 48
- N. Sourial, H. Bergman, S. Karunananthan, et al.
Implementing frailty into clinical practice: A cautionary tale
J Gerontol A Biol Sci Med Sci, 68 (2013), pp. 1505-1511
- 49
- C. Beaudart, R. Rizzoli, O. Bruyere, et al.
Sarcopenia: Burden and challenges for public health
Arch Public Health, 72 (2014), p. 45
- 50
- I. Janssen, D.S. Shepard, P.T. Katzmarzyk, R. Roubenoff
The healthcare costs of sarcopenia in the United States
J Am Geriatr Soc, 52 (2004), pp. 80-85
- 51
- J.O. Bock, H.H. Konig, H. Brenner, et al.
Associations of frailty with health care costs - results of the ESTHER cohort study
BMC Health Serv Res, 16 (2016), p. 128
- 52
- S. Gariballa, A. Alessa
Sarcopenia: Prevalence and prognostic significance in hospitalized patients
Clin Nutr, 32 (2013), pp. 772-776
- 53
- S. Joglekar, P.N. Nau, J.J. Mezhir
The impact of sarcopenia on survival and complications in surgical oncology: A review of the current literature
J Surg Oncol, 112 (2015), pp. 503-509
- 54
- P. Abizanda, A. Sinclair, N. Barcons, et al.
Costs of malnutrition in institutionalized and community-dwelling older adults: A systematic review
J Am Med Dir Assoc, 17 (2016), pp. 17-23
- 55
- S. von Haehling
The wasting continuum in heart failure: From sarcopenia to cachexia
Proc Nutr Soc, 74 (2015), pp. 1-11
- 56
- P. Stenvinkel, J.J. Carrero, F. von Walden, et al.
Muscle wasting in end-stage renal disease promulgates premature death: established, emerging and potential novel treatment strategies
Nephrol Dial Transplant (2015)
- 57
- J.M. Argiles
Cancer-associated malnutrition
Eur J Oncol Nurs, 9 (2005), pp. S39-S50
- 58
- J.M. Argiles, S. Busquets, B. Stemmler, F.J. Lopez-Soriano
Cancer cachexia: Understanding the molecular basis
Nat Rev Cancer, 14 (2014), pp. 754-762
- 59
- J.M. Argiles, C.C. Fontes-Oliveira, M. Toledo, et al.
Cachexia: A problem of energetic inefficiency
J Cachexia Sarcopenia Muscle, 5 (2014), pp. 279-286
- 60
- C.E. Baldwin, A.D. Bersten
Myopathic characteristics in septic mechanically ventilated patients
Curr Opin Clin Nutr Metab Care, 18 (2015), pp. 240-247
- 61
- C. Guillet, Y. Boirie
Insulin resistance: A contributing factor to age-related muscle mass loss?
Diabetes Metab, 31 (2005)
5S20–5S26
- 62
- B.P. Martinez, A.K. Batista, I.B. Gomes, et al.
Frequency of sarcopenia and associated factors among hospitalized elderly patients
BMC Musculoskelet Disord, 16 (2015), p. 108
- 63
- W.J. Evans, J.E. Morley, J. Argiles, et al.
Cachexia: A new definition
Clin Nutr, 27 (2008), pp. 793-799
- 64
- A. Kalinkovich, G. Livshits
Sarcopenia—The search for emerging biomarkers
Ageing Res Rev, 22 (2015), pp. 58-71
- 65
- A.J. Cruz-Jentoft, F. Landi, S.M. Schneider, et al.
Prevalence of and interventions for sarcopenia in ageing adults: A systematic review. Report of the International Sarcopenia Initiative (EWGSOP and IWGS)
Age Ageing, 43 (2014), pp. 748-759
- 66
- N.E. Deutz, J.M. Bauer, R. Barazzoni, et al.
Protein intake and exercise for optimal muscle function with aging: Recommendations from the ESPEN Expert Group
Clin Nutr, 33 (2014), pp. 929-936
- 67
- E. Arentson-Lantz, S. Clairmont, D. Paddon-Jones, et al.
Protein: A nutrient in focus
Appl Physiol Nutr Metab, 40 (2015), pp. 755-761
- 68
- R. Calvani, A. Miccheli, F. Landi, et al.
Current nutritional recommendations and novel dietary strategies to manage sarcopenia
J Frailty Aging, 2 (2013), pp. 38-53
- 69
- J. Bauer, G. Biolo, T. Cederholm, et al.
Evidence-based recommendations for optimal dietary protein intake in older people: A position paper from the PROT-AGE Study Group
J Am Med Dir Assoc, 14 (2013), pp. 542-559
- 70
- A.C. Milne, J. Potter, A. Vivanti, A. Avenell
Protein and energy supplementation in elderly people at risk from malnutrition
Cochrane Database Syst Rev (2) (2009), p. CD003288
- 71
- M. Girón, J. Vílchez, R. Salto, et al.
Conversion of leucine to β-hydroxy-β-methylbutyrate by α-keto isocaproate dioxygenase is required for a potent stimulation of protein synthesis in L6 rat myotubes
J Cachexia, Sarcopenia, and Muscle, 7 (2016), pp. 68-78
- 72
- D.J. Wilkinson, T. Hossain, D.S. Hill, et al.
Effects of leucine and its metabolite beta-hydroxy-beta-methylbutyrate on human skeletal muscle protein metabolism
J Physiol, 591 (2013), pp. 2911-2923
- 73
- S.L. Casperson, M. Sheffield-Moore, S.J. Hewlings, D. Paddon-Jones
Leucine supplementation chronically improves muscle protein synthesis in older adults consuming the RDA for protein
Clin Nutr, 31 (2012), pp. 512-519
- 74
- M. Leenders, L.B. Verdijk, L. van der Hoeven, et al.
Prolonged leucine supplementation does not augment muscle mass or affect glycemic control in elderly type 2 diabetic men
J Nutr, 141 (2011), pp. 1070-1076
- 75
- S. Verhoeven, K. Vanschoonbeek, L.B. Verdijk, et al.
Long-term leucine supplementation does not increase muscle mass or strength in healthy elderly men
Am J Clin Nutr, 89 (2009), pp. 1468-1475
- 76
- T. Alon, D. Bagchi, H.G. Preuss
Supplementing with beta-hydroxy-beta-methylbutyrate (HMB) to build and maintain muscle mass: A review
Res Commun Mol Pathol Pharmacol, 111 (2002), pp. 139-151
- 77
- N.E. Deutz, S.L. Pereira, N.P. Hays, et al.
Effect of beta-hydroxy-beta-methylbutyrate (HMB) on lean body mass during 10 days of bed rest in older adults
Clin Nutr, 32 (2013), pp. 704-712
- 78
- P.J. Fitschen, G.J. Wilson, J.M. Wilson, K.R. Wilund
Efficacy of beta-hydroxy-beta-methylbutyrate supplementation in elderly and clinical populations
Nutrition, 29 (2013), pp. 29-36
- 79
- S.R. Kimball, L.S. Jefferson
Signaling pathways and molecular mechanisms through which branched-chain amino acids mediate translational control of protein synthesis
J Nutr, 136 (2006), pp. 227S-231S
- 80
- S. Nissen, R. Sharp, M. Ray, et al.
Effect of leucine metabolite beta-hydroxy-beta-methylbutyrate on muscle metabolism during resistance-exercise training
J Appl Physiol (1985), 81 (1996), pp. 2095-2104
- 81
- S.M. Pasiakos, L.M. Margolis, J.S. Orr
Optimized dietary strategies to protect skeletal muscle mass during periods of unavoidable energy deficit
FASEB J, 29 (2015), pp. 1136-1142
- 82
- S. Portal, A. Eliakim, D. Nemet, et al.
Effect of HMB supplementation on body composition, fitness, hormonal profile and muscle damage indices
J Pediatr Endocrinol Metab, 23 (2010), pp. 641-650
- 83
- K.A. Szczesniak, P. Ostaszewski, J.C. Fuller Jr., et al.
Dietary supplementation of beta-hydroxy-beta-methylbutyrate in animals—a review
J Anim Physiol Anim Nutr (Berl), 99 (2015), pp. 405-417
- 84
- G.J. Wilson, J.M. Wilson, A.H. Manninen
Effects of beta-hydroxy-beta-methylbutyrate (HMB) on exercise performance and body composition across varying levels of age, sex, and training experience: A review
Nutr Metab (Lond), 5 (2008), p. 1
- 85
- M. Van Koevering, S. Nissen
Oxidation of leucine and alpha-ketoisocaproate to beta-hydroxy-beta-methylbutyrate in vivo
Am J Physiol, 262 (1992), pp. E27-E31
- 86
- S. Baier, D. Johannsen, N. Abumrad, et al.
Year-long changes in protein metabolism in elderly men and women supplemented with a nutrition cocktail of beta-hydroxy-beta-methylbutyrate (HMB), L-arginine, and L-lysine
J Parenter Enteral Nutr, 33 (2009), pp. 71-82
- 87
- M.D. Giron, J.D. Vilchez, S. Shreeram, et al.
beta-Hydroxy-beta-methylbutyrate (HMB) normalizes dexamethasone-induced autophagy-lysosomal pathway in skeletal muscle
PLoS One, 10 (2015), p. e0117520
- 88
- L.A. Burton, D. Sumukadas
Optimal management of sarcopenia
Clin Interv Aging, 5 (2010), pp. 217-228
- 89
- H.J. Denison, C. Cooper, A.A. Sayer, S.M. Robinson
Prevention and optimal management of sarcopenia: A review of combined exercise and nutrition interventions to improve muscle outcomes in older people
Clin Interv Aging, 10 (2015), pp. 859-869
- 90
- J.E. Morley
Pharmacologic options for the treatment of sarcopenia
Calcif Tissue Int, 94 (2016), pp. 319-333
- 91
- B.F. Miller, J.L. Olesen, M. Hansen, et al.
Coordinated collagen and muscle protein synthesis in human patella tendon and quadriceps muscle after exercise
J Physiol, 567 (2005), pp. 1021-1033
- 92
- E. Pasini, S. Le Douairon Lahaye, V. Flati, et al.
Effects of treadmill exercise and training frequency on anabolic signaling pathways in the skeletal muscle of aged rats
Exp Gerontol, 47 (2012), pp. 23-28
- 93
- T. Wenz, S.G. Rossi, R.L. Rotundo, et al.
Increased muscle PGC-1alpha expression protects from sarcopenia and metabolic disease during aging
Proc Natl Acad Sci U S A, 106 (2009), pp. 20405-20410
- 94
- T.S. Bowen, G. Schuler, V. Adams
Skeletal muscle wasting in cachexia and sarcopenia: Molecular pathophysiology and impact of exercise training
J Cachexia Sarcopenia Muscle, 6 (2015), pp. 197-207
- 95
- K. Dideriksen, S. Reitelseder, L. Holm
Influence of amino acids, dietary protein, and physical activity on muscle mass development in humans
Nutrients, 5 (2013), pp. 852-876