7.5: Photosynthesis
- Page ID
- 88936
\( \newcommand{\vecs}[1]{\overset { \scriptstyle \rightharpoonup} {\mathbf{#1}} } \)
\( \newcommand{\vecd}[1]{\overset{-\!-\!\rightharpoonup}{\vphantom{a}\smash {#1}}} \)
\( \newcommand{\id}{\mathrm{id}}\) \( \newcommand{\Span}{\mathrm{span}}\)
( \newcommand{\kernel}{\mathrm{null}\,}\) \( \newcommand{\range}{\mathrm{range}\,}\)
\( \newcommand{\RealPart}{\mathrm{Re}}\) \( \newcommand{\ImaginaryPart}{\mathrm{Im}}\)
\( \newcommand{\Argument}{\mathrm{Arg}}\) \( \newcommand{\norm}[1]{\| #1 \|}\)
\( \newcommand{\inner}[2]{\langle #1, #2 \rangle}\)
\( \newcommand{\Span}{\mathrm{span}}\)
\( \newcommand{\id}{\mathrm{id}}\)
\( \newcommand{\Span}{\mathrm{span}}\)
\( \newcommand{\kernel}{\mathrm{null}\,}\)
\( \newcommand{\range}{\mathrm{range}\,}\)
\( \newcommand{\RealPart}{\mathrm{Re}}\)
\( \newcommand{\ImaginaryPart}{\mathrm{Im}}\)
\( \newcommand{\Argument}{\mathrm{Arg}}\)
\( \newcommand{\norm}[1]{\| #1 \|}\)
\( \newcommand{\inner}[2]{\langle #1, #2 \rangle}\)
\( \newcommand{\Span}{\mathrm{span}}\) \( \newcommand{\AA}{\unicode[.8,0]{x212B}}\)
\( \newcommand{\vectorA}[1]{\vec{#1}} % arrow\)
\( \newcommand{\vectorAt}[1]{\vec{\text{#1}}} % arrow\)
\( \newcommand{\vectorB}[1]{\overset { \scriptstyle \rightharpoonup} {\mathbf{#1}} } \)
\( \newcommand{\vectorC}[1]{\textbf{#1}} \)
\( \newcommand{\vectorD}[1]{\overrightarrow{#1}} \)
\( \newcommand{\vectorDt}[1]{\overrightarrow{\text{#1}}} \)
\( \newcommand{\vectE}[1]{\overset{-\!-\!\rightharpoonup}{\vphantom{a}\smash{\mathbf {#1}}}} \)
\( \newcommand{\vecs}[1]{\overset { \scriptstyle \rightharpoonup} {\mathbf{#1}} } \)
\( \newcommand{\vecd}[1]{\overset{-\!-\!\rightharpoonup}{\vphantom{a}\smash {#1}}} \)
\(\newcommand{\avec}{\mathbf a}\) \(\newcommand{\bvec}{\mathbf b}\) \(\newcommand{\cvec}{\mathbf c}\) \(\newcommand{\dvec}{\mathbf d}\) \(\newcommand{\dtil}{\widetilde{\mathbf d}}\) \(\newcommand{\evec}{\mathbf e}\) \(\newcommand{\fvec}{\mathbf f}\) \(\newcommand{\nvec}{\mathbf n}\) \(\newcommand{\pvec}{\mathbf p}\) \(\newcommand{\qvec}{\mathbf q}\) \(\newcommand{\svec}{\mathbf s}\) \(\newcommand{\tvec}{\mathbf t}\) \(\newcommand{\uvec}{\mathbf u}\) \(\newcommand{\vvec}{\mathbf v}\) \(\newcommand{\wvec}{\mathbf w}\) \(\newcommand{\xvec}{\mathbf x}\) \(\newcommand{\yvec}{\mathbf y}\) \(\newcommand{\zvec}{\mathbf z}\) \(\newcommand{\rvec}{\mathbf r}\) \(\newcommand{\mvec}{\mathbf m}\) \(\newcommand{\zerovec}{\mathbf 0}\) \(\newcommand{\onevec}{\mathbf 1}\) \(\newcommand{\real}{\mathbb R}\) \(\newcommand{\twovec}[2]{\left[\begin{array}{r}#1 \\ #2 \end{array}\right]}\) \(\newcommand{\ctwovec}[2]{\left[\begin{array}{c}#1 \\ #2 \end{array}\right]}\) \(\newcommand{\threevec}[3]{\left[\begin{array}{r}#1 \\ #2 \\ #3 \end{array}\right]}\) \(\newcommand{\cthreevec}[3]{\left[\begin{array}{c}#1 \\ #2 \\ #3 \end{array}\right]}\) \(\newcommand{\fourvec}[4]{\left[\begin{array}{r}#1 \\ #2 \\ #3 \\ #4 \end{array}\right]}\) \(\newcommand{\cfourvec}[4]{\left[\begin{array}{c}#1 \\ #2 \\ #3 \\ #4 \end{array}\right]}\) \(\newcommand{\fivevec}[5]{\left[\begin{array}{r}#1 \\ #2 \\ #3 \\ #4 \\ #5 \\ \end{array}\right]}\) \(\newcommand{\cfivevec}[5]{\left[\begin{array}{c}#1 \\ #2 \\ #3 \\ #4 \\ #5 \\ \end{array}\right]}\) \(\newcommand{\mattwo}[4]{\left[\begin{array}{rr}#1 \amp #2 \\ #3 \amp #4 \\ \end{array}\right]}\) \(\newcommand{\laspan}[1]{\text{Span}\{#1\}}\) \(\newcommand{\bcal}{\cal B}\) \(\newcommand{\ccal}{\cal C}\) \(\newcommand{\scal}{\cal S}\) \(\newcommand{\wcal}{\cal W}\) \(\newcommand{\ecal}{\cal E}\) \(\newcommand{\coords}[2]{\left\{#1\right\}_{#2}}\) \(\newcommand{\gray}[1]{\color{gray}{#1}}\) \(\newcommand{\lgray}[1]{\color{lightgray}{#1}}\) \(\newcommand{\rank}{\operatorname{rank}}\) \(\newcommand{\row}{\text{Row}}\) \(\newcommand{\col}{\text{Col}}\) \(\renewcommand{\row}{\text{Row}}\) \(\newcommand{\nul}{\text{Nul}}\) \(\newcommand{\var}{\text{Var}}\) \(\newcommand{\corr}{\text{corr}}\) \(\newcommand{\len}[1]{\left|#1\right|}\) \(\newcommand{\bbar}{\overline{\bvec}}\) \(\newcommand{\bhat}{\widehat{\bvec}}\) \(\newcommand{\bperp}{\bvec^\perp}\) \(\newcommand{\xhat}{\widehat{\xvec}}\) \(\newcommand{\vhat}{\widehat{\vvec}}\) \(\newcommand{\uhat}{\widehat{\uvec}}\) \(\newcommand{\what}{\widehat{\wvec}}\) \(\newcommand{\Sighat}{\widehat{\Sigma}}\) \(\newcommand{\lt}{<}\) \(\newcommand{\gt}{>}\) \(\newcommand{\amp}{&}\) \(\definecolor{fillinmathshade}{gray}{0.9}\)Plants have evolved different photosynthetic adaptations, called C3, CAM and C4. All are essentially the reverse of respiration. We’ll consider each in turn later. Chemically, photosynthesis is the reverse reaction of respiration. Compare the following chemical reactions of respiration and photosynthesis:
If respiration (reaction 1) is a complete oxidation of glucose to \(\rm H_2O\) and \(\rm CO_2\), then photosynthesis (reaction 2) is a reduction of \(\rm CO_2\) by electrons from \(\rm H_2O\) to make glucose. Thus, photosynthesis is an endergonic reaction pathway. During photosynthesis, sunlight (i.e., visible light) fuels the reduction of \(\rm CO_2\) (Figure 7.4).

Photosynthesis began in the absence of oxygen; it preceded oxygenic respiration on Earth. But after it evolved, it led to increasing levels of atmospheric oxygen that resulted in the selection of oxygenic respiratory pathways (the Krebs cycle, electron transport, and oxidative phosphorylation). We will see here that photosynthesis and respiration both have electrontransport-ATP-synthesizing systems that share similar features. This suggests that both pathways share a common evolutionary ancestry. Elsewhere, we will consider what a common ancestral system might have looked like. Two biochemical pathways make up photosynthesis:
- Light-dependent reactions use visible light energy to remove electrons from water to reduce electron carriers, to pump protons, and to make ATP.
- Light-independent reactions use ATP to transfer electrons from the reduced electron carriers to \(\rm CO_2\) to synthesize glucose.
These light-dependent and light-independent pathways of photosynthesis are summarized below in Figure. 7.5.

7.5.1. The Light-Dependent Reactions
Colored substances contain pigments which reflect the colors that we see, and which simultaneously absorb all the other colors of visible light. Early studies asked which plant pigments absorbed the light that allow (or as we say now, “support”) photosynthesis. The abundant chlorophyll pigment we see in plant tissues is actually two separate green pigments: chlorophyll a and chlorophyll b. One might therefore predict that light absorbed by either or both chlorophylls will support photosynthesis. Figure 7.6 illustrates the experiment that tested this hypothesis.

The action spectrum of photosynthesis (Figure 7.7) plots the results of this experiment.
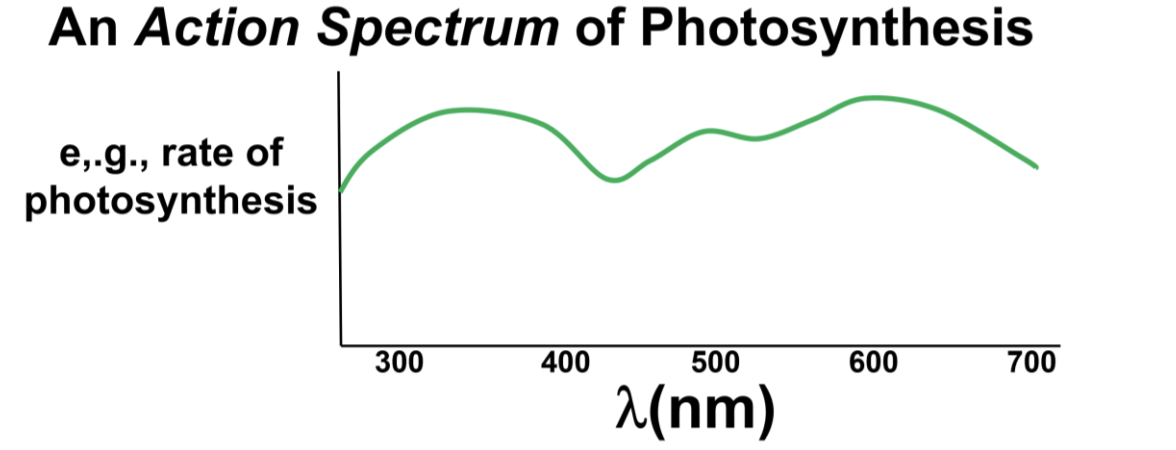
The spectrum shows that all wavelengths of visible light energy can support photosynthesis. In addition, other experiments revealed that radiation other than visible light (e.g., ultraviolet and infrared light) do not support photosynthesis. One can conclude that chlorophylls are likely not the only pigments to support photosynthesis.
Chlorophylls are easily purified from leaves. The graph in Figure 7.8 shows an average absorbance spectrum of chlorophylls. The absorbance of chlorophyll a and chlorophyll b are slightly different, but they center at wavelengths of 450 nm and 675 nm.

Comparing the action and absorbance spectra, we can conclude that the chlorophylls support photosynthesis, but that chlorophylls alone do not account for the action spectrum of photosynthesis!
In fact, other pigments absorbing light elsewhere in the visible spectrum also support photosynthesis. Of course, we knew that these other pigments were present in leaves and other photosynthetic plant tissues because we could see many of them as fall colors. All of these pigments (including chlorophylls) are found in chloroplasts, the organelles that conduct photosynthesis in plants. Take another look at the structure of chloroplasts in the electron micrographs in Figure 7.9.

Figure 7.10 shows that the visible light absorbance spectra of the chlorophylls, carotenoids and possibly other pigments coincide with the photosynthetic action spectrum. This implies that the absorption of light by those pigments is responsible for photosynthesis.

In fact, chlorophyll a, chlorophyll b, carotenoids, and other accessory pigments participate in capturing light energy for photosynthesis. Two pigment clusters, called reaction centers, capture light energy as part of photosystem 1 (PSI) and photosystem 2 (PSII) on thylakoid membranes of chloroplasts.
Johann Deisenhofer, Robert Huber and Hartmut Michel first determined the 3D structure of a bacterial reaction center. Then they unraveled the relationship between the structure of the proteins in the center and the membrane in which they were embedded. For this, they shared the 1988 Nobel Prize in Chemistry.
The activities of PSI are animated at Photosystem I Action. You can watch as photon of light excites electron (\(\rm e^{−}\)) pairs from PSI pigments, which then transfer their energy from pigment to pigment, ultimately to chlorophyll a P700. The impact of the electron pair excites a pair of electrons from chlorophyll a P700, and that pair is captured by a PSI \(\rm e^{−}\) acceptor, an event referred to as charge separation. Next, the reduced PSI acceptor is oxidized as electrons move down a short ETC, eventually reducing \(\rm NADP^{+}\) to NADPH. Electrons on NADPH will eventually be used to reduce \(\rm CO_2\) to a carbohydrate. So far, so good! But that leaves an electron deficit in PSI. The Z-Scheme illustrated in Figure 7.11 follows electrons taken from water (absorbed through roots) into PSII, which will replace those electrons missing from PSI.

Let’s summarize electron flow from water through the Z-scheme. Light excites an \(\rm e^{−}\) pair from the P680 form of chlorophyll a in PSII. A PSII electron acceptor in the thylakoid membrane, identified as pheophytin, captures these electrons, another act of photosynthetic charge separation. An electron transport chain oxidizes the pheophytin, transferring \(\rm e^{−}\) pairs down to PSI.
Some of the free energy released then pumps protons from the stroma into the luminal space of the thylakoid membranes. The gradient free energy fuels ATP synthesis as protons flow back into the stroma through a chloroplast ATP synthase. The video at Z-Scheme Action animates the entire Z-Scheme, showing first how PSI electrons reduce \(\rm NADP^{+}\) and then how PSII electrons replace missing PSI electrons, making ATP along the way. The oxygen released by splitting water ends up in the atmosphere.
To help you get a handle on the Z-scheme, list all the products of the light reactions of photosynthesis.
The goal of photosynthesis is to make and to store glucose, so the photosynthesizing cells get ATP to power glucose production by photophosphorylation during electron transport via the Z-scheme. However, cyclic photophosphorylation can occur when the cell’s need for ATP exceeds the capacity of the Z-scheme to supply it. Cyclic photophosphorylation is illustrated in Figure 7.12.

Cyclic Photophosphorylation is a variation on the light-dependent reactions, a kind of time-out to make ATP without reducing \(\rm NADP^{+}\) and thus, without reducing \(\rm CO_2\) to make sugar). The cycle simply takes excited electrons to the PSI electron acceptor, and instead of sending them to \(\rm NADP^{+}\), it deposits them on PC (plastocyanin) in the electron transport chain between PSII and PSI. These electrons then flow down the “long line” of the Z, right back to PSI, releasing their free energy to make ATP. In light, the electrons just go up and around, hence the name Cyclic Photophosphorylation. The path of electrons shown in Figure 7.12 is also animated at Cyclic Photophosphorylation Activity.
7.5.2. The Light-Independent ("Dark") Reactions of Photosynthesis
As we have seen, the light-dependent reactions of photosynthesis require light energy and water to generate \(\rm O_2\), ATP, and NADPH. In the light-independent (or “dark”) reactions of chloroplasts, the ATP and NADPH will provide free energy and electrons (respectively) for carbon fixation (the reduction of \(\rm CO_2\) to make carbohydrates). There are three main pathways for the so-called “dark reactions.”
7.5.2.a The Calvin-Cycle C3 Pathway
The Calvin cycle is the most common light-independent reaction pathway. In 1950 Melvin Calvin and his colleagues (James Bassham and Andrew Benson) described the complete cycle from \(\rm CO_2\) to glucose (Figure 7.13). They followed the radioactive \({}^{14} \rm C\) isotope of carbon into \({}^{14} \rm CO_2\), then into glucose, and then into carbohydrates in photosynthesizing plants. For his work, Calvin received the Nobel Prize in Chemistry in 1961.

Why is the C3 photosynthetic pathway called C3?
Check the animation at Calvin Cycle Action. Each carbon dioxide entering the Calvin cycle is “fixed” to a 5-C ribulose bisphosphate molecule (RuBP), catalyzed by the enzyme RuBP carboxylase-oxygenase, or RUBISCO for short. Seemingly, the expected 6-C molecule quickly splits into two 3-C molecules since it has not been found as an intermediate to date! The first detectable products are two molecules of 3PGA (3-phosphoglyceric acid). Each 3PGA is in turn reduced to glyceraldehyde-3-phosphate (GA3P in the illustration, or G-3- P). Some of the Calvin cycle intermediates should look familiar: the cycle regenerates the RuBP and the essential intermediates of glucose production. Figure 7.14 may help with the arithmetic of the Calvin cycle.

Perhaps the easiest way to see this is to imagine the cycle going around six times, fixing six molecules of \(\rm CO_2\), as shown in the illustration. In fact, a photosynthetic cell must fix six \(\rm CO_2\) carbons onto six RuBP molecules to generate a single 6-C glucose. This leaves thirty carbons on the table (as G-3-P molecules) with which to make six 5-C RuBP molecules.
In 2020, scientists engineered artificial chloroplasts based on spinach photosynthetic cells. The artificial chloroplasts used light to fix CO2 even more efficiently than the real things (A Better Chloroplast)! What does this tell you about evolution?
There are times that even plants in temperate environments suffer prolonged hot, dry spells. Perhaps you have seen a lawn grow more slowly and turn brown after a dry heat wave in summer, only to grow and re-green after the rains resume. C3 plants resort to photorespiration during drought and dry weather, closing their stomata to conserve water. Under these conditions, \(\rm CO_2\) can’t get into the leaves—and \(\rm O_2\) can’t get out! As \(\rm CO_2\) levels drop and \(\rm O_2\) levels rise in photosynthetic cells, the Calvin cycle slows down. Instead of fixing \(\rm CO_2\), the enzyme RUBISCO now catalyzes “\(\rm O_2\) fixation” using its oxygenase activity. The combination of RuBP with \(\rm O_2\) splits RuBP into a three-carbon and a two-carbon molecule: 3- phosphoglyceric acid (3-PG) and phosphoglycolate, respectively (Figure 7.15).

Under these conditions, not only does photorespiration result in only one three-carbon carbohydrate (compared to two in the Calvin cycle), but the phosphoglycolate produced is cytotoxic (not healthy for cells!). Removing phosphate and metabolizing the remaining glycolic acid costs energy. Therefore, photorespiration can only be sustained for a short time.
On the other hand, plants that have adapted to live in hot, arid environments all the time have evolved one of two alternate pathways: the CAM (Crassulacean Acid Metabolism) and the C4 pathway. Each is an alternative to the C3 pathway of carbon fixation.
7.5.2.b The CAM Photosynthetic Pathway
Crassulacean acid metabolism (CAM) was discovered in the Crassulaceae family, which contains succulents like sedum (a common ground cover), cactuses, jade plants, and some orchids. This pathway was selected in evolution to allow plants to conserve water, especially during the high daytime temperatures.
The CAM pathway is shown below in Figure 7.16. Stomata in chlorenchyma (mesophyll leaf cells) close during the day to minimize water loss, which would result from transpiration. The stomata open at night, allowing plant tissues to take up \(\rm CO_2\). CAM plants fix \(\rm CO_2\) by combining it with PEP (phosphoenolpyruvate) to make oxaloacetate (OAA). This eventually produces malic acid that is stored in plant cell vacuoles.

By day, stored malic acid retrieved from the vacuoles splits into pyruvate and \(\rm CO_2\). The \(\rm CO_2\) then enters chloroplasts and joins the Calvin cycle to make glucose and the starches. In sum, CAM plant mesophyll cells do the following:
- They open stomata at night to collect, to fix, and to store \(\rm CO_2\) as an organic acid.
- They close stomata to conserve water in the daytime.
- They re-fix the stored \(\rm CO_2\) as carbohydrates, using the NADPH and ATP from the light reactions the next the day.
7.5.2.c The C4 Photosynthetic Pathway
The end-product of the C4 pathway is the malic acid (4 carbons). As in the CAM pathway, phosphoenolpyruvate (PEP) carboxylase catalyzes carbon fixation, converting PEP to oxaloacetate (OAA) and OAA to malate. But the pathways differ in what next happens to malate (malic acid). Figure 7.17 (below) shows the role of malate in the C4 pathway.

C4 metabolism diverges from the CAM pathway after malate formation. PEP-carboxylase catalysis is rapid in C4 plants, in part because malic acid does not accumulate in the mesophyll cells. Instead, it is rapidly transferred from mesophyll to adjacent bundle sheath cells, where it enters chloroplasts. As a result, the C4 plants can keep their stomata open to capture \(\rm CO_2\) for part of the day (unlike CAM plants), but they must close them for at least part of the day to conserve water. The four-carbon malic acid is oxidized to pyruvate (three carbons) in the bundle-sheath-cell chloroplasts. The released \(\rm CO_2\) enters the Calvin cycle to be rapidly fixed by RUBISCO. Of course, this system allows more efficient water use and faster carbon fixation under dry, hot conditions than does C3 photosynthesis. Corn is perhaps the best-known C4 plant!
Thinking about the economies of evolution, can you recognize several other intermediates common to respiration and the light-independent photosynthetic reactions?